النبات
مواضيع عامة في علم النبات
الجذور - السيقان - الأوراق
النباتات الوعائية واللاوعائية
البذور (مغطاة البذور - عاريات البذور)
الطحالب
النباتات الطبية
الحيوان
مواضيع عامة في علم الحيوان
علم التشريح
التنوع الإحيائي
البايلوجيا الخلوية
الأحياء المجهرية
البكتيريا
الفطريات
الطفيليات
الفايروسات
علم الأمراض
الاورام
الامراض الوراثية
الامراض المناعية
الامراض المدارية
اضطرابات الدورة الدموية
مواضيع عامة في علم الامراض
الحشرات
التقانة الإحيائية
مواضيع عامة في التقانة الإحيائية
التقنية الحيوية المكروبية
التقنية الحيوية والميكروبات
الفعاليات الحيوية
وراثة الاحياء المجهرية
تصنيف الاحياء المجهرية
الاحياء المجهرية في الطبيعة
أيض الاجهاد
التقنية الحيوية والبيئة
التقنية الحيوية والطب
التقنية الحيوية والزراعة
التقنية الحيوية والصناعة
التقنية الحيوية والطاقة
البحار والطحالب الصغيرة
عزل البروتين
هندسة الجينات
التقنية الحياتية النانوية
مفاهيم التقنية الحيوية النانوية
التراكيب النانوية والمجاهر المستخدمة في رؤيتها
تصنيع وتخليق المواد النانوية
تطبيقات التقنية النانوية والحيوية النانوية
الرقائق والمتحسسات الحيوية
المصفوفات المجهرية وحاسوب الدنا
اللقاحات
البيئة والتلوث
علم الأجنة
اعضاء التكاثر وتشكل الاعراس
الاخصاب
التشطر
العصيبة وتشكل الجسيدات
تشكل اللواحق الجنينية
تكون المعيدة وظهور الطبقات الجنينية
مقدمة لعلم الاجنة
الأحياء الجزيئي
مواضيع عامة في الاحياء الجزيئي
علم وظائف الأعضاء
الغدد
مواضيع عامة في الغدد
الغدد الصم و هرموناتها
الجسم تحت السريري
الغدة النخامية
الغدة الكظرية
الغدة التناسلية
الغدة الدرقية والجار الدرقية
الغدة البنكرياسية
الغدة الصنوبرية
مواضيع عامة في علم وظائف الاعضاء
الخلية الحيوانية
الجهاز العصبي
أعضاء الحس
الجهاز العضلي
السوائل الجسمية
الجهاز الدوري والليمف
الجهاز التنفسي
الجهاز الهضمي
الجهاز البولي
المضادات الحيوية
مواضيع عامة في المضادات الحيوية
مضادات البكتيريا
مضادات الفطريات
مضادات الطفيليات
مضادات الفايروسات
علم الخلية
الوراثة
الأحياء العامة
المناعة
التحليلات المرضية
الكيمياء الحيوية
مواضيع متنوعة أخرى
الانزيمات
Electron Microscopy
المؤلف:
D. DeRosier
المصدر:
Nature 357, 196–199
الجزء والصفحة:
2-5-2016
2661
Electron Microscopy
High-resolution electron microscopy presents to the molecular biologist advantages and disadvantages compared to both X-ray crystallography and NMR. The advantages are that (i) the macromolecule of interest need not necessarily be highly purified, provided that it can be unambiguously recognized, (ii) only small quantities (mg rather than mg amounts) are required, (iii( even very large macromolecules or complexes with no symmetry can be imaged easily, (iv( specimens can be imaged in their natural environment, and (v) unlike X-ray diffraction patterns, images contain both structure factor amplitudes and phases. The major disadvantages are that (i) the contrast of biological preparations in the electron microscope is inherently low, (ii) the specimen must be imaged in a high vacuum, and (iii) biological material is easily damaged by electron radiation. Electron microscopy has developed to the state where it can complement X-ray crystallography and NMR; this is especially true in the case of cryoelectron microscopy of frozen specimens with the application of computer image processing techniques. An example of how all three techniques may complement each other was the structural characterization of dihydrolipoyl transacetylase (1-3). X-ray crystallography provided the rigid core structure. NMR provided information about the flexible domains of this molecule, and electron microscopy integrated both packets of information into a detailed model.
The transmission electron microscope (TEM) is the electron counterpart to the transmission light microscope. A high-velocity, homogeneous electron beam passes through a specimen and is imaged on a phosphorescent screen or a charge-coupled device (CCD). The mechanism of image formation, however, for the TEM is by scattering, which is quite different from that of the light microscope, which is by absorption. TEMs are divided into different classes, depending on the accelerating voltages. A conventional TEM (CTEM) accelerates electrons with typical voltages between 60 and 120 kV; an intermediate-high voltage TEM (IVEM) operates between 200 and 400 kV; and a high-voltage TEM (HVEM) operates above 500 kV. The principal advantages of higher voltage TEMs are (i) the capability of imaging thicker specimens, and (ii) increased resolution of biological crystals and ice-embedded particles.
1. Thin-Section CTEM
The vast majority of biological specimens are much too thick as they occur in nature to be penetrated by the electron beam. A CTEM allows for quality imaging of specimens generally no thicker than 200 nm. Hence, the requirement for thin sections of biological material that are able to withstand both the high vacuum in the electron microscope and electron irradiation is apparent. Electron microscopy of thin sections of cells and tissues provides in situ visualization of cellular morphology and the spatial relationships of subcellular components. In order to preserve detailed structural information, the tissue must be well-fixed, dehydrated and embedded in plastic. The resolution achieved with tissue prepared this way is usually limited to 2–3 nm. Sections from the embedded tissue should be of a known and uniform thickness and free from breaks, folds, wrinkles, and chatter to produce standardized and reproducible observations (4). Because it is difficult to differentiate among the electron opacity of various cellular components, selective staining is necessary. Commonly used stains are heavy metals such as uranyl acetate and lead salts.
2. Freeze Fracturing and Etching
Thin-section techniques can be complemented by examining specimen surfaces by freeze fracturing/etching. The freeze fracture method involves the following steps: (i) cryofixation of the specimen by rapid freezing, (ii) fracturing of the specimen by cleaving it with a knife edge or breaking it, (iii) replication of the freeze-fracture plane by coating with heavy metals under high vacuum, (iv) removal of the specimen from the replica, and (v) observation of the replicas with a CTEM. The most important contribution of this technique has been the elucidation of the molecular architecture of biological membranes. During freeze fracturing, membranes are split through the hydrophobic moiety, thus exposing intramembraneous surfaces. The fracture plane often follows the contours of membranes and leaves either protrusions or depressions where it passes around organelles or vesicles (4). Smooth areas represent the lipid monolayer face, whereas particles represent integral membrane proteins.
Freeze etching is a slight modification of freeze fracturing through the process of controlled sublimation of water vapor (transition from the solid state to the gas state, bypassing the fluid state( to expose membrane surfaces or macromolecules just below the fracture plane. By etching, extracellular and intracellular structures, such as the cytoskeleton, can be visualized which would otherwise be masked. The etching can either be “normal” (< ~ 100 nm) or “deep” (up to ~ 3 µm.(
Related techniques involve shadowing of macromolecules adsorbed or deposited onto a flat surface and dried by methods which minimized deformation during drying. Drying is best accomplished by sublimation as in freeze-etching and requires access to a freeze-fracture instrument; this is referred to as freeze drying and shadowing (5). A simpler method developed by Branton requires only a conventional vacuum evaporator (6). This technique involves (i) preparing the macromolecule in a high-concentration glycerol solution, (ii) spraying this solution onto mica, (iii) evaporating the solvent under vacuum, (iv) rotary shadowing with a metal such as platinum, (v) carbon coating the shadowed specimen, (vi) floating the carbon replica onto water, and (vii) picking up the replica on an electron microscope grid. Rotary shadowing is particularly useful for visualizing fibers or rod-shaped complexes such as actin/myosin. The double strands of spectrin (which forms rods ~200 nm long) were resolved using this technique with tantalum/tungsten shadowing, which produced small metal grains that increase the resolution (7).
References
1. D. DeRosier (1992) Nature 357, 196–199.
2. A. Mattevi et al. (1992) Science 255, 1544–1547.
3.M. A. Robin et al. (1992) Biochemistry 31, 3463–3471.
4. M. A. Hayat (1989) Principles and Techniques of Electron Microscopy, CRC Press, Boca Raton, FL.
5. J. Kistler, U. Aebi, and M. Kellenberger (1977) J. Ultrastruct. Res. 59, 76–86.
6. J. M. Tyler and D. Branton (1980) J. Ultrastruct. Res. 71, 95–102.
7. J. R. Glenney (1987) In Electron Microscopy in Molecular Biology (J. Sommerville and U. Scheer, eds.), IRL Press, Oxford, pp. 167–178.
الاكثر قراءة في مواضيع عامة في الاحياء الجزيئي
اخر الاخبار
اخبار العتبة العباسية المقدسة
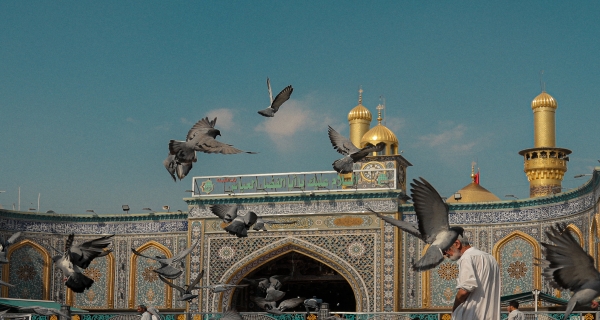
الآخبار الصحية
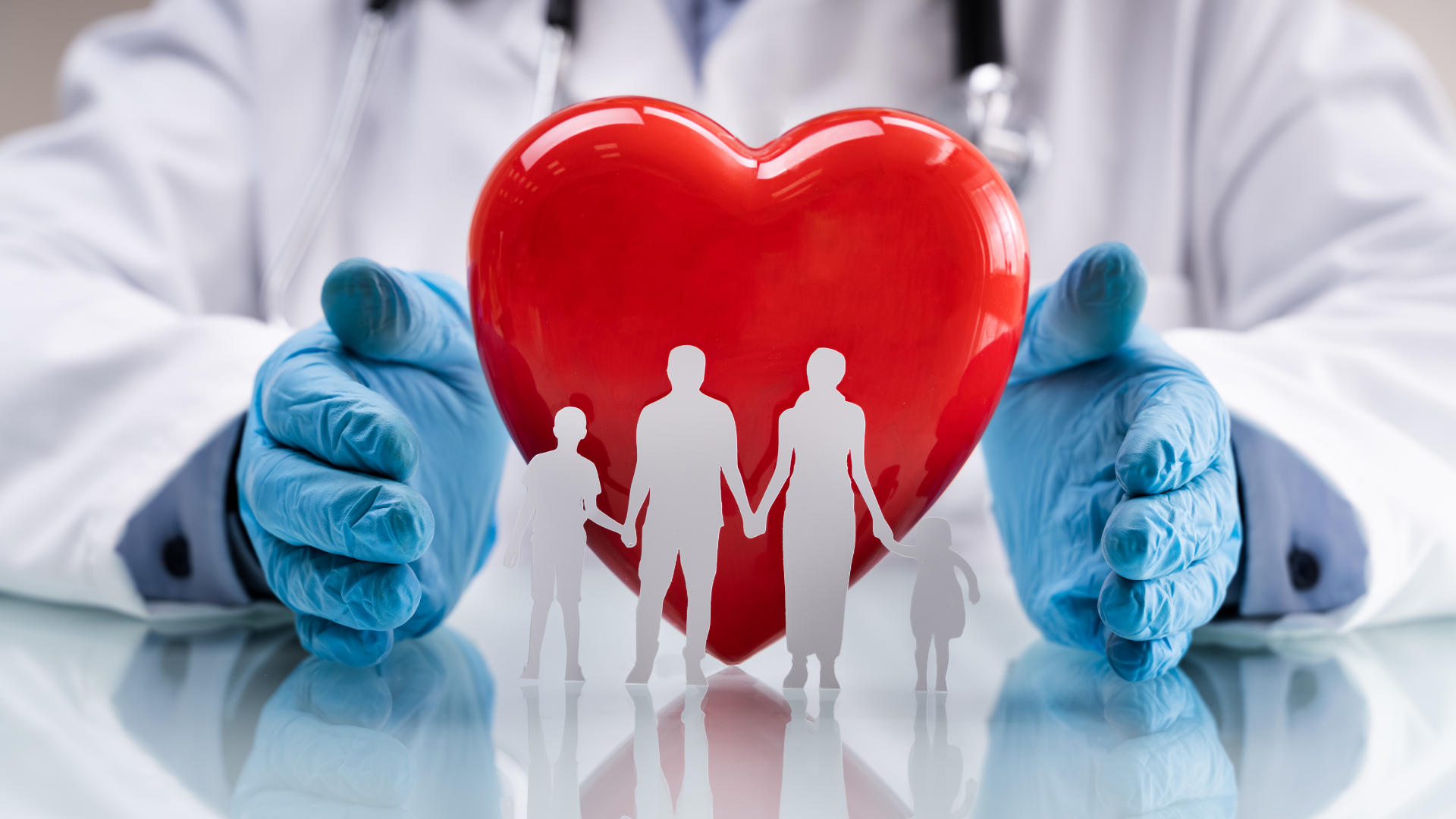