النبات
مواضيع عامة في علم النبات
الجذور - السيقان - الأوراق
النباتات الوعائية واللاوعائية
البذور (مغطاة البذور - عاريات البذور)
الطحالب
النباتات الطبية
الحيوان
مواضيع عامة في علم الحيوان
علم التشريح
التنوع الإحيائي
البايلوجيا الخلوية
الأحياء المجهرية
البكتيريا
الفطريات
الطفيليات
الفايروسات
علم الأمراض
الاورام
الامراض الوراثية
الامراض المناعية
الامراض المدارية
اضطرابات الدورة الدموية
مواضيع عامة في علم الامراض
الحشرات
التقانة الإحيائية
مواضيع عامة في التقانة الإحيائية
التقنية الحيوية المكروبية
التقنية الحيوية والميكروبات
الفعاليات الحيوية
وراثة الاحياء المجهرية
تصنيف الاحياء المجهرية
الاحياء المجهرية في الطبيعة
أيض الاجهاد
التقنية الحيوية والبيئة
التقنية الحيوية والطب
التقنية الحيوية والزراعة
التقنية الحيوية والصناعة
التقنية الحيوية والطاقة
البحار والطحالب الصغيرة
عزل البروتين
هندسة الجينات
التقنية الحياتية النانوية
مفاهيم التقنية الحيوية النانوية
التراكيب النانوية والمجاهر المستخدمة في رؤيتها
تصنيع وتخليق المواد النانوية
تطبيقات التقنية النانوية والحيوية النانوية
الرقائق والمتحسسات الحيوية
المصفوفات المجهرية وحاسوب الدنا
اللقاحات
البيئة والتلوث
علم الأجنة
اعضاء التكاثر وتشكل الاعراس
الاخصاب
التشطر
العصيبة وتشكل الجسيدات
تشكل اللواحق الجنينية
تكون المعيدة وظهور الطبقات الجنينية
مقدمة لعلم الاجنة
الأحياء الجزيئي
مواضيع عامة في الاحياء الجزيئي
علم وظائف الأعضاء
الغدد
مواضيع عامة في الغدد
الغدد الصم و هرموناتها
الجسم تحت السريري
الغدة النخامية
الغدة الكظرية
الغدة التناسلية
الغدة الدرقية والجار الدرقية
الغدة البنكرياسية
الغدة الصنوبرية
مواضيع عامة في علم وظائف الاعضاء
الخلية الحيوانية
الجهاز العصبي
أعضاء الحس
الجهاز العضلي
السوائل الجسمية
الجهاز الدوري والليمف
الجهاز التنفسي
الجهاز الهضمي
الجهاز البولي
المضادات الحيوية
مواضيع عامة في المضادات الحيوية
مضادات البكتيريا
مضادات الفطريات
مضادات الطفيليات
مضادات الفايروسات
علم الخلية
الوراثة
الأحياء العامة
المناعة
التحليلات المرضية
الكيمياء الحيوية
مواضيع متنوعة أخرى
الانزيمات
Electron Crystallography
المؤلف:
R. Henderson
المصدر:
Quarterly Rev. Biophys.
الجزء والصفحة:
1-5-2016
2516
Electron Crystallography
Knowledge on the molecular basis of many biological processes has rapidly evolved as a result of the development of complementary techniques of molecular biology and high-resolution structural biology. Quite often the mode of action of biological macromolecules can only be understood at the atomic level. Thousands of protein structures have been solved to atomic scale resolution using X-ray crystallography, but only a handful by electron crystallography. However, electron microscopy is a technique intrinsically capable of atomic resolution. The transmission electron microscope itself can achieve resolution on the order of 0.2–0.3 nm, and the structures of small organic and inorganic molecules are routinely determined at atomic dimensions. It is now well established that the principal limitation to obtaining atomic resolution of biological specimens using electron microscopy is the specimen itself (1). Because biological molecules are sensitive to electron radiation, one must record electron micrographs of unstained specimens with very low levels of electron irradiation (this is called low-dose imaging) in order to preserve high-resolution information. Unfortunately, such low electron doses yield images with a very low signal-to-noise ratio, too low to observe any structural detail. High signal-to-noise ratio images can be obtained by averaging hundreds or thousands of low-dose images of identical structures (2). Two-dimensional crystals, thin crystals which are only one unit cell thick along one axis, currently offer the best way to average images of many molecules to build up the signal-to-noise at atomic or near-atomic resolution (3, 4). It was estimated that the average of ~5 million molecules was required to produce a 3-D density map of bacteriorhodopsin that had recognizable amino acid side chains (1, 5). Similarly, ~2.6 million molecules of light-harvesting complex II were averaged to obtain an equivalent resolution (6).
Electron crystallography encompasses the use of electron diffraction (7) and imaging of thin crystals suitable for the electron microscope. All electron images are 2-D projections of a 3-D object along the path of the electron beam. Hence, to reconstruct an object in 3-D it is necessary to collect images from different angles. The minimal number of tilted views needed to reconstruct an object at a given resolution can be calculated from geometrical considerations (8).
Once a 2-D or thin 3-D crystal is grown, the best way to evaluate its quality is to study its diffraction pattern. The quickest and easiest method is to exam electron micrographs by optical diffraction. In this technique, an electron micrograph is illuminated with laser light focused with a series of lenses to form an optical diffraction pattern showing the distribution of Fourier transform intensities in the same manner as an X-ray diffraction pattern of a 3-D crystal. The spacing and distribution of intensities in an optical diffraction pattern offers clues to the molecular packing and secondary structure (9). Optical diffraction is also an important step in judging image quality in terms of astigmatism, defocus, and drift. More information can be obtained from the computed diffraction produced by calculating the Fourier transform of the digitized image. Unlike X-ray diffraction patterns, the computed diffraction pattern will have both structure factor amplitudes and phases in the “weak phase object approximation” (thin object and sufficiently high electron energy). Hence, a reconstruction of the object can be calculated from the Fourier transform of the image using the structure factor amplitudes and phases of the crystal; this results in a reconstructed image of the average unit cell of the crystal with noise removed. Furthermore, the phase relationship of the symmetry-related spots in the computed diffraction pattern can be used to determine the crystallographic symmetry.
Diffraction patterns, similar in principle to those obtained in X-ray diffraction, are formed in the back focal plane of the objective lens in the electron microscope. By adjusting the current in the diffraction lens, the diffraction pattern can be observed on the viewing screen. The type of pattern observed depends on the arrangement of molecules in the crystal and on how well ordered they are. How the molecules are arranged within the repeating unit of a crystal (unit cell) can be determined by the symmetry of the diffraction pattern and the intensities of the diffraction spots. In addition to the molecular arrangement, diffraction patterns can reveal the degree of specimen preservation and show the distribution of ordered regions of the specimen. The further the spots extend, the higher is the resolution of the preserved detail. Diffraction patterns can be used to assess radiation damage to the specimen with increasing electron dose, as judged by fading of the higher-order diffraction spots. The size of crystals most amenable for electron diffraction of biological macromolecules is a few microns on edge and one unit cell thick, usually described as a 2-D crystal (10). However, the electron diffraction patterns of 3-D crystals can also be examined if they are less than a few hundred Angstroms thick (11); Perkins, unpublished results). Electron diffraction can also ascertain the effects of different methods of specimen preparation. If a protein has secondary structural elements aligned in parallel, as in fibers, the spacings of the diffraction spots or streaks can indicate if there is predominantly a-helix or b-sheet (12). One disadvantage of electron diffraction is that relatively large crystals (~ 100 × 100 unit cells) are needed before high-resolution spots are observed. Another disadvantage is that like X-ray diffraction, electron diffraction does not produce structure factor phases; hence the structure cannot be determined from electron diffraction alone (13).
For high-resolution structural studies, advances in imaging, computer image processing, and preservation of specimens without negative stain and fixative have been critically important. The native conformation of specimens must be preserved under high vacuum conditions, and this can be achieved using one of two types of embedment media: sugars (14-16) and vitreous ice (17), which have both been shown to preserve the protein crystal structure well enough to produce images at a resolution of 0.3 nm. In addition, the development of computer-controlled spot-scan imaging has improved the efficiency of transfer of high resolution image information (18). Improvement in the rate of data acquisition has been made using cameras employing a cooled charge-coupled device (19). Because the high-resolution signal-to-noise is low, averaging over several large (20) or many more small (4) images is necessary to restore the signal above the background. Despite several noteworthy breakthroughs, the rate of progress in determining high-resolution structures of biological macromolecules by electron microscopy has been slowed by the paucity of suitable, well-ordered crystals. Recent reviews have described crystallization methods for both water-soluble and membrane proteins (21-23). Perhaps further improvements in collecting high-resolution information from single particles will obviate the need for crystals (1).
Electron crystallography offers a viable alternative to X-ray crystallography when crystals are not large enough for X-ray diffraction or for membrane proteins (24). Integral membrane proteins have the advantage that they can be routinely reconstituted in their native environment, the lipid bilayer, and often can be forced into ordered arrays through the judicious choice of lipid/protein and detergent/protein concentrations. The first membrane protein structure revealed by electron crystallography was bacteriorhodopsin in which seven a-helices were resolved (25). Since this early work, the field of electron crystallography has advanced to the point that atomic models have been built for two membrane proteins (5, 6, 26) and good progress made toward this goal for several others (27-29). Tubulin crystals also diffract to high resolution, and electron crystallography has shown the location of the taxol-binding site (30, 31). The highest resolution structures have been generated by taking the phases from the images and the amplitudes from the electron diffraction patterns. The electron diffraction amplitudes are generally more accurate than the image amplitudes because the image amplitudes require correction for instrumental factors that affect image quality, and methods for this correction have not been fully developed (20). However, it is not always necessary for electron microscopy to be performed at high resolution to contribute to atomic resolution structures. Electron microscopy provided a critical link allowing the actin crystal structure determined by X-ray diffraction to be fit into the actin filament determined by electron microscopy (32, 33) .
The structure of biological macromolecules with helical or icosahedral symmetries can be determined using the same underlying principles of electron crystallography, although the operational approaches will be different (34). Helical structures produce diffraction patterns in which the helical information is confined to regularly spaced layerlines perpendicular to the helix axis instead of discrete spots. The pattern of intensities along the layerlines usually produce X-shaped patterns. Helical symmetry provides different views of a molecule in a single image as identical molecules are arranged along a helical path, so in principle only one image is needed for a low-resolution 3-D reconstruction. As with electron crystallography, however, higher resolution requires many images in order to obtain views at many different angles and to average many images in order to improve the signal-to-noise ratio (35). The structures of helical assemblies have been solved to 0.9 nm resolution (35, 36), and higher resolution is shortly anticipated. Close to 0.7 nm resolution has been reported for a viral structure with icosahedral symmetry (37). Even with the benefits of high symmetry elements afforded by an icosahedron, more than 6000 images needed to be averaged to achieve this resolution.
References
1. R. Henderson (1995) Quarterly Rev. Biophys. 28, 171–193.
2. R. M. Glaeser and K. H. Downing (1993) Ultramicroscopy 52, 478–486.
3. D. Brillinger et al. (1989) J. Applied Stat. 16, 165–175.
4. G. A. Perkins, K. H. Downing, and R. M. Glaeser (1995) Ultramicroscopy 60, 283–294.
5. R. Henderson et al. (1990) J. Mol. Biol. 213, 899–929.
6. W. Kuhlbrandt, D. N. Wang, and Y. Fujiyoshi (1994) Nature 367, 614–621.
7. D. L. Misell and E. B. Brown (1987) Electron Diffraction: An Introduction for Biologists, Elsevier, Amsterdam, The Netherlands.
8.A. Klug (1979) Chem. Scr. 14, 245–256.
9. F. Thon (1971) In Electron Microscopy in Material Science (U. Valdre, ed.), Academic Press, New York, pp. 571–625.
10. T. A. Ceska and R. Henderson (1990) J. Mol. Biol. 213, 539–560.
11. T. W. Jeng et al. (1984) J. Mol. Biol. 175, 93–97.
12. R. D. B. Fraser and T. P. MacRae (1973) Conformation in Fibrous Proteins, Academic Press, New York.
13. J. M. Baldwin and R. Henderson (1984) Ultramicroscopy 14, 319–335.
14. P. N. T. Unwin and R. Henderson (1975) J. Mol. Biol. 94, 425–440.
15. W. Kuhlbrandt (1988) J. Mol. Biol. 202, 849–864.
16. G. Perkins et al. (1993) J. Microscopy 169, 61–65.
17. J. Dubochet et al. (1988) Quarterly Rev. Biophys. 21, 129–228.
18.K. H. Downing (1991) Science 251, 53–59.
19. A. J. Koster et al. (1992) Ultramicroscopy 46, 207–228.
20. J. M. Baldwin et al. (1988) J. Mol. Biol. 202, 585–591.
21. R. Kornberg and S. A. Darst (1991) Curr. Opin. Struct. Biol. 1, 642–646.
22. B. K. Jap et al. (1992) Ultramicroscopy 46, 45–84.
23. W. Kuhlbrandt (1992) Quarterly Rev. Biophys. 25, 1–49.
24. A. Engel et al. (1992) J. Struct. Biol. 109, 219–234.
25. R. Henderson and P. N. T. Unwin (1975) Nature 257, 28–32.
26. N. Grigorieff et al. (1996) J. Mol. Biol. 259, 393–421.
27. A. Olofsson, V. Mallouh, and A. Brisson (1994) J. Struct. Biol. 113, 199–205.
28. H. Hebert, I. Schmidt-Krey, and R. Morgenstern (1995) EMBO J. 14, 3864–3869.
29. T. Walz et al. (1995) Nature Struct. Biol. 2, 730–732.
30. E. Nogales et al. (1995) Nature 375, 424–427.
31. S. G. Wolf et al. (1996) J. Mol. Biol. 262, 485–501.
32. W. Kabsch et al. (1990) Nature 347, 37–44.
33. K. C. Holmes et al. (1992) In Mechanism of Myofilament Sliding in Muscle Contraction (H. Sugi and G. H. Pollack, eds.), Plenum Press, New York.
34. D. G. Morgan and D. De Rosier (1992) Ultramicroscopy 46, 263–286.
35. T. W. Jeng et al. (1989) J. Mol. Biol. 205, 251–257.
36. D. G. Morgan et al. (1995) J. Mol. Biol. 249, 88–110.
37. B. Bottcher, S. A. Wynne, and R. A. Crowther (1997) Nature 386, 88–91.
الاكثر قراءة في مواضيع عامة في الاحياء الجزيئي
اخر الاخبار
اخبار العتبة العباسية المقدسة
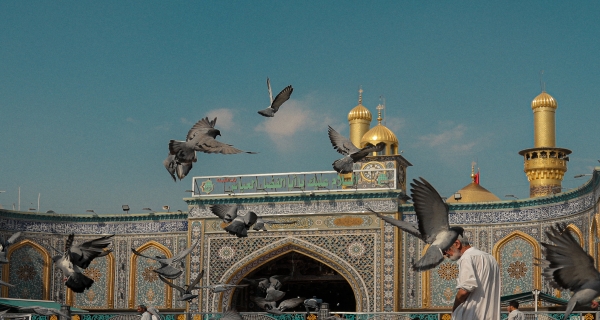
الآخبار الصحية
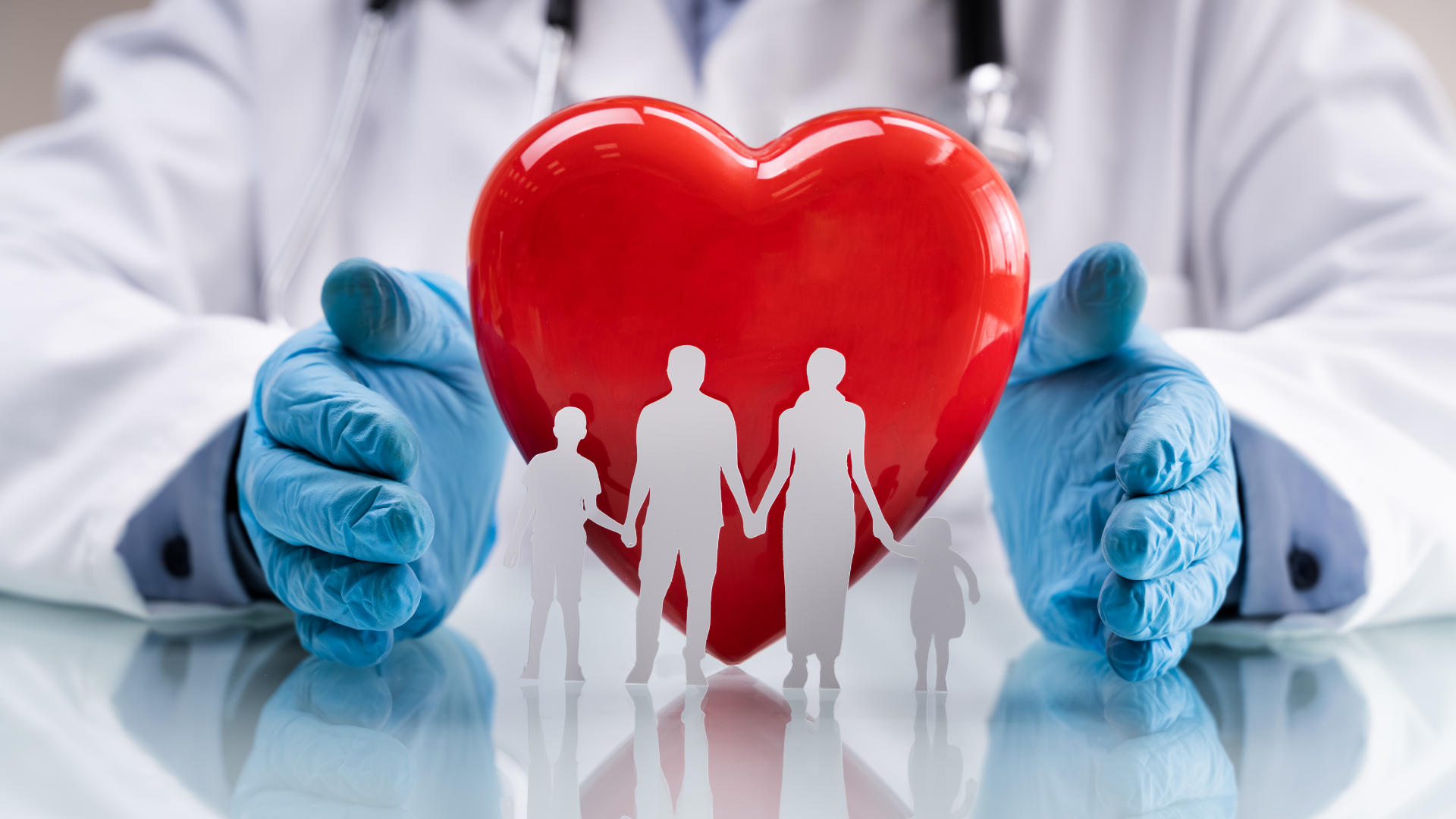