النبات
مواضيع عامة في علم النبات
الجذور - السيقان - الأوراق
النباتات الوعائية واللاوعائية
البذور (مغطاة البذور - عاريات البذور)
الطحالب
النباتات الطبية
الحيوان
مواضيع عامة في علم الحيوان
علم التشريح
التنوع الإحيائي
البايلوجيا الخلوية
الأحياء المجهرية
البكتيريا
الفطريات
الطفيليات
الفايروسات
علم الأمراض
الاورام
الامراض الوراثية
الامراض المناعية
الامراض المدارية
اضطرابات الدورة الدموية
مواضيع عامة في علم الامراض
الحشرات
التقانة الإحيائية
مواضيع عامة في التقانة الإحيائية
التقنية الحيوية المكروبية
التقنية الحيوية والميكروبات
الفعاليات الحيوية
وراثة الاحياء المجهرية
تصنيف الاحياء المجهرية
الاحياء المجهرية في الطبيعة
أيض الاجهاد
التقنية الحيوية والبيئة
التقنية الحيوية والطب
التقنية الحيوية والزراعة
التقنية الحيوية والصناعة
التقنية الحيوية والطاقة
البحار والطحالب الصغيرة
عزل البروتين
هندسة الجينات
التقنية الحياتية النانوية
مفاهيم التقنية الحيوية النانوية
التراكيب النانوية والمجاهر المستخدمة في رؤيتها
تصنيع وتخليق المواد النانوية
تطبيقات التقنية النانوية والحيوية النانوية
الرقائق والمتحسسات الحيوية
المصفوفات المجهرية وحاسوب الدنا
اللقاحات
البيئة والتلوث
علم الأجنة
اعضاء التكاثر وتشكل الاعراس
الاخصاب
التشطر
العصيبة وتشكل الجسيدات
تشكل اللواحق الجنينية
تكون المعيدة وظهور الطبقات الجنينية
مقدمة لعلم الاجنة
الأحياء الجزيئي
مواضيع عامة في الاحياء الجزيئي
علم وظائف الأعضاء
الغدد
مواضيع عامة في الغدد
الغدد الصم و هرموناتها
الجسم تحت السريري
الغدة النخامية
الغدة الكظرية
الغدة التناسلية
الغدة الدرقية والجار الدرقية
الغدة البنكرياسية
الغدة الصنوبرية
مواضيع عامة في علم وظائف الاعضاء
الخلية الحيوانية
الجهاز العصبي
أعضاء الحس
الجهاز العضلي
السوائل الجسمية
الجهاز الدوري والليمف
الجهاز التنفسي
الجهاز الهضمي
الجهاز البولي
المضادات الحيوية
مواضيع عامة في المضادات الحيوية
مضادات البكتيريا
مضادات الفطريات
مضادات الطفيليات
مضادات الفايروسات
علم الخلية
الوراثة
الأحياء العامة
المناعة
التحليلات المرضية
الكيمياء الحيوية
مواضيع متنوعة أخرى
الانزيمات
Cytokinins
المؤلف:
M. Strnad, W. Peters, E. Beck, and M. Kamínek
المصدر:
Plant Physiol. 99, 74–80
الجزء والصفحة:
31-12-2015
3603
Cytokinins
1. History
The first discovery that plants make compounds that stimulate cell division dates back to 1913, when Haberlandt (1) demonstrated that an unknown substance present in vascular tissues of various plants caused cork cambium formation and wound healing in cut potato tubers. These molecules were later called cytokinins, from the Greek “cytokinesis” (cell division). In the 1940s, van Overbeek et al. (2( showed that similar compounds were also present in the milky endosperm of immature coconuts. In ,1954 Miller et al. (3) isolated a substance, formed by partial breakdown of herring sperm DNA, that, in synergy with auxin, stimulated cell division in tobacco pith cells very actively. The molecule was called kinetin (6-furfurylamino purine). Trans-zeatin (6-(4-hydroxy-3-methylbut-trans-2-enylamino) purine) was the first cytokinin to be identified from maize endosperm (4) (Fig. 1).
Figure 1. Structural formula of trans-zeatin
2. Biosynthesis and Metabolism
Natural cytokinins (CKs) are primarily purine derivatives with an N6-substituted isopentenylated side chain (iP-type CKs) (5). However, a series of CKs with an aromatic side chain (eg, benzyl-adenine) have been demonstrated in certain species (6, 7). The most abundant naturally occurring CKs are CK nucleosides and CK nucleotides (5). Certain phenolic compounds also possess cell-division-promoting activity, but are not classified as true cytokinins (8).
The origin of CKs in plants remains a subject of debate. Roots are the primary sites of cytokinin biosynthesis, although limited synthesis in the shoot has also been demonstrated (9, 10). CKs have been proposed to be transported from root to shoot by the transpiration stream in xylem tissues, but conclusive evidence is lacking (11). Even though present throughout the plant, CKs have never been proven to be synthesized by endogenous, plant-borne enzymes. Because many root-associated bacteria are cytokinin producers, it has been hypothesized that CKs are made exclusively by these microbial symbionts (12).
Cytokinins can be synthesized via a direct (de novo) or an indirect (transfer RNA) pathway. The main source of free CKs is the de novo pathway. The key step is the formation of N6-(D2-isopentenyl) adenosine-5′-monophosphate from D2-isopentenyl pyrophosphate and AMP catalyzed by isopentenyltransferase. The isopentenyl side chain originates from mevalonate, which also serves as a precursor to abscisic acid, gibberellins, and brassinosteroids. Isopentenyltransferase was first characterized from bacteria and slime molds (5, 7). Different constructs using the Agrobacterium isopentenyltransferase IPT (tmr) gene were used to generate constitutive or controlled cytokinin overproduction in transgenic plants (13-15). These studies mainly confirmed the classical view of cytokinin action in plants. Specific manipulation of senescence was achieved by placing the IPT gene under the control of a senescence-specific promoter (16). Recently, isopentenyltransferase activity was also demonstrated in plants (17), but the enzyme was never purified to homogeneity, nor have plant genes encoding isopentenyltransferase been cloned. This is the major reason why CK production by the plant itself is still questioned. An Arabidopsis mutant with sixfold elevated CK levels was isolated from a screen for seedlings with multiple cotyledons (18, 19). The phenotype of this amp1 (altered meristem program1) mutant is reminiscent of several CK effects, including enhanced lateral branching, elevated rate of leaf formation, induction of flowering and floral morphological abnormalities, and delayed senescence. In addition, amp1 displays a de-etiolated phenotype in the dark and is an allele of cop2 (constitutive photomorphogenic 2). It was proposed that CK induces light-regulated genes in a light-independent fashion (19). The AMP1 gene product may be required for CK degradation, or it could be a negative regulator of CK biosynthesis. A second mutant cri (cristal) that accumulates sixfold more CK than the wild type was recently identified (20). Cri1 mutants are phenotypically different from amp1 and show symptoms of vitrification.
The second biosynthetic route for CKs is via tRNA. Modified bases in tRNA, including isopentenylated bases, have been found in virtually all organisms investigated (21). CK modification in tRNA is always at the adenosine residue immediately 3′ to the anticodon of tRNAs that read codons starting with U (5). These CK residues in tRNA may serve as a direct, albeit not major, precursor of free CK, as a result of tRNA turnover. The rate of CK release in this way is dependent on the metabolic activity of the cell. The major CK in tRNA is cis-zeatin, with very low CK activity, but it can be converted to the biologically active trans isomer (Fig. 1) by a cis–trans isomerase (22 (.
Several modifications can occur to the N6-substituted side chain and purine ring. Formation of zeatin and its nucleoside and nucleotide derivatives, most probably by hydroxylation of the isopentenyl adenine moiety, is common in most plant tissues. The hydroxylase that mediates this conversion was isolated from cauliflower (23). O-Glycosylation of hydroxylated CKs is often observed, but leads to a considerable decrease in activity (5). CK-O-glucosides can be converted to free active CK by the action of b-glucosidases. In contrast, modification of the purine ring by N-glucosylation of the 3, 7, or 9 position yields inactive, stable derivatives that cannot generate free CKs. N-Glucosylation is thus expected to play an important role in regulating the levels of active CKs. An additional control is exerted by CK oxidase (24, 25). This enzyme catalyzes degradation of the N6-(D2-isopentenyl( side chain and irreversibly leads to loss of CK activity. CK nucleotides are not accepted as a substrate. A general role for CK oxidase is to contribute to CK homeostasis in plants (26).
3. Signal Perception and Transduction
A number of CK-binding proteins have been described, one of which is a potential CK receptor (27, 28). The latter is a cytosolic protein that reversibly binds zeatin and is involved in transcriptional activation in the presence of zeatin, but not in its absence. Only the trans isomer elicited the observed effect.
Two candidate plasma membrane CK receptors were identified by mutational analysis (29, 30). Mutants of Arabidopsis that exhibit shoot formation in tissue culture in the absence of CK were obtained by an activation-tagging approach. The CKI1 (cytokinin-independent 1) gene was isolated and encodes a protein similar to two-component regulators of bacteria (29). As in the case of the ethylene receptor ETR1, CKI1 is a hybrid kinase with fused sensor and receiver domains. Based on this analogy and on the fact that constitutive overexpression of CKI1 results in typical effects of CK action, CKI1 is hypothesized to encode a CK receptor. Overexpression would enable cells to sense endogenous concentrations below the threshold, which normally leads to CK response. Intriguingly, phytochromes also display similarities with two-component systems. It has been speculated that they might regulate other two-component systems, thus enabling cross-talk between the ethylene and CK pathways (31). A second candidate CK receptor is a seven-transmembrane domain receptor (7TM), characteristic in G-protein signaling and involved in transduction of extracellular signals (30). The Arabidopsis GCR1 (G-coupled receptor 1) gene was isolated by polymerase chain reaction (PCR), and antisense mutagenesis with a 35S aGCR1 construct resulted in reduced cotyledon and leaf expansion and a single flowering stem, a phenotype reminiscent of the cyr1 (cytokinin resistant 1) mutant. However, GCR1 and CYR1 do not represent the same gene (30).
The above findings confirm the involvement of both phosphorylation and G proteins in CK signaling (32) . Sense and antisense expression in tobacco of a gene encoding a small GTP-binding protein from rice resulted in a dwarf phenotype and abnormal flower development, correlating with a sixfold elevation of CK levels compared to wild type (33). Phosphorylation plays an important role in cell cycle control by CK (32). In addition, evidence was given for involvement of intracellular Ca+ in CK signaling (see Calcium Signaling) mostly from studies in mosses (32). Finally, the similarity of a CK-binding protein to S-adenosyl-homocysteine hydrolase raised the possibility that some CK effects might be mediated through control of methylation of DNA and/or proteins (34).
Further progress in understanding CK Signal Transduction is expected from mutant studies. Several CK-resistant mutants have been identified, mainly in Arabidopsis (35). Five loci have been studied in detail. The majority were isolated using a root elongation response in the presence of CK. In certain cases, resistance to other hormones was found. For instance, the ckr1 (cytokinin resistant 1( mutant is allelic to ein2 (ethylene-insensitive 2), indicating cross-talk with the ethylene pathway. It was demonstrated that the inhibition of root elongation by CK results from an induction of ethylene biosynthesis (36). This was exploited by screening for the absence of triple response in the dark, in the presence of kinetin (37). The cin5 (cytokinin-insensitive 5) mutant affects the 1-aminocyclopropane-1-carboxylate synthase gene 5 in Arabidopsis. Disruption of the carboxyl terminus of the same isoform leads to ethylene overproduction, previously identified as an ethylene overproducer (eto2) mutant. A third class of CK–resistant mutants is cyr1 (38). In contrast to ckr1 and cin5, cyr1 displays an abnormal shoot phenotype: cotyledons and leaves fail to expand, a limited number of leaves are formed, and a single infertile flower is made. No resistance to other hormones was observed; however, cyr1 is hypersensitive to abscisic acid (38). Cyr1 is probably allelic to emf2 (embryonic flower 2). EMF gene products might function in the maintenance of vegetative growth (39) .The stp1 (stunted plant 1) mutant has a reduced sensitivity to CKs, both in assays for elongation and radial swelling of the root (40). The general morphology was not affected, but a reduction in growth rate was observed. The function of the STP1 gene product remains to be elucidated. Finally, a recent study describes identification of three loci involved in control of cell division and plant development (41). The corresponding mutants were called pasticcino (pas) and showed hypertrophy of their apical parts when grown on CK-containing medium, a phenotype reminiscent of abnormal shoots regenerated on media with unbalanced auxin/CK ratio and strikingly similar to the fasciation disease caused by Rhodococcus fascians (41). The altered embryo, root, and leaf development result from uncoordinated cell divisions. PAS genes are hypothesized to play a role in CK signaling, because no differences in CK levels were found compared to wild type.
4. Downstream Targets
Primary CK response genes have not yet been isolated, nor have CK responsive cis-acting elements or trans-acting factors been identified (42). A large number of genes that are either induced or repressed upon CK treatment have been described. Controls are exerted at a transcriptional or post-transcriptional level. Changes in gene expression were observed between 2 h and 48 h after treatment. Many of these secondary CK response genes are involved in light regulation and nutrition (32, 42) .
5. Effects
Depending on temporal and spatial factors, a particular subset of downstream target genes is activated by CK treatment and results in one of many described effects. Cytokinins regulate many aspects of plant growth and development, including cell division, enlargement, and differentiation, as well as chloroplast development, release of apical dominance, nutrient mobilization, flowering, and delay of senescence (43, 44).
References
1. G. Haberlandt (1913) Sitzungsber. K. Preuss. Akad. Wissensch., 318–345.
2. J. van Overbeek, M. E. Conklin, and A. F. Blakeslee (1941) Science 94, 350–351.
3. C. O. Miller, F. Skoog, M. H. Von Saltza, and F. Strong (1955) J. Am. Chem. Soc. 77, 1392–1393.
4. D. S. Letham (1963) Life Sci. 8, 569–573.
5. C.-m. Chen (1997) Physiol. Plant. 101, 665–673.
6. M. Strnad, W. Peters, E. Beck, and M. Kamínek (1992) Plant Physiol. 99, 74–80.
7. E. Prinsen, M. Kamínek, and H. Van Onckelen (1997) Plant Growth Regul. 23, 3–15.
8. R. A. Teutonico, M. W. Dudley, J. D. Orr, D. G. Lynn, and A. N. Binns (1991) Plant Physiol. 297-288.
9. A. Carmi, and J. Van Staden (1983) Plant Physiol. 73, 76–78.
10. C.-M. Chen, J. R. Ertl, S. M. Leisner, and C.-C. Chang (1985) Plant Physiol. 78, 510–513.
11. P. D. Hare, W. A. Cress, and J. van Staden (1997) Plant Growth Regul. 23, 79–103.
12. M. A. Holland (1997) Plant Physiol. 115, 865–868.
13. J. I. Medford, R. Horgan, Z. El-Sawi, and H. J. Klee (1989) Plant Cell 1, 403–413.
14. T. Schmülling, S. Beinsberger, J. De Greef, J. Schell, H. A. Van Onckelen, and A. Spena (1989( FEBS Lett. 249, 401–406.
15. J. J. Estruch, E. Prinsen, H. Van Onckelen, J. Schell, and A. Spena (1991) Science 254, 1364–1367.
16. S. Gan and R. M. Amasino (1997) Plant Physiol. 113, 313–319.
17. J. R. Blackwell and R. Horgan (1994) Phytochemistry 35, 339–342.
18. A. M. Chaudhury, S. Letham, S. Craig, and E. S. Dennis (1993) Plant J. 4, 907–916.
19. A. N. Chin-Atkins, S. Craig, C. H. Hocart, E. S. Dennis, and A. M. Chaudhury (1996) Planta 198,549-556.
20. V. Santoni, M. Delarue, M. Caboche, and C. Bellini (1997) Planta 202, 62–69.
21. N. Murai (1981) In Cytokinins: Chemistry, Activity and Function (D. W. S. Mok and M. C. Mok, eds.), CRC Press, Boca Raton, FL, pp. 87–99.
22. N. V. Bassil, D. W. S. Mok, and M. C. Mok (1993) Plant Physiol. 102, 867–872.
23. C.-m. Chen and S. M. Leisner (1984) Plant Physiol. 75, 442–446.
24. D. J. Armstrong (1994) In Cytokinins: Chemistry, Activity, and Function (D. W. S. Mok and M. C. Mok, eds.), CRC Press, Boca Raton, FL, pp. 139–154.
25. R. J. Jones and B. M. N. Schreiber (1997) Plant Growth Regul. 23, 123–134.
26. S. Eklöf, C. Astot, T. Moritz, J. Blackwell, O. Olsson, and G. Sandberg (1996) Physiol. Plant. 98, 333-344.
27. C. Brinegar (1994) In Cytokinins: Chemistry, Activity, and Function (D. W. S. Mok and M. C. Mok, eds.), CRC Press, Boca Raton, FL, pp. 217–232.
28. O. N. Kulaeva, N. N. Karavaiko, S. Y. Selivankina, Y. V. Zemlyachenko, and S. V. Shipilova (1995) FEBS Lett. 366, 26–28.
29. T. Kakimoto (1996) Science 274, 982–985.
30. S. Plakidou-Dymock, D. Dymock, and R. Hooley (1998) Curr. Biol. 8, 315–324.
31. J. W. Reed (1998) Trends Plant Sci. 3, 43–44.
32. P. D. Hare, and J. van Staden (1997) Plant Growth Regul. 23, 41–78.
33. H. Sano, S. Deo, E. Orudgev, S. Youssefian, K. Isizuka, and Y. Ohashi (1994) Proc. Natl. Acad. Sci. USA 91, 10556–10560.
34. S. Mitsui, T. Wakasugi, and M. Sugiura (1996) Plant Growth Regul. 18, 39–43.
35. J. Deikman (1997) Plant Growth Regul. 23, 33–40.
36. A. J. Cary, W. Liu, and S. H. Howell (1995) Plant Physiol. 107, 1075–1082.
37. J. P. Vogel, K. E. Woeste, A. Theologis, and J. J. Kieber (1998) Proc. Natl. Acad. Sci. USA 9547711-4766, .
38. J. Deikman and M. Ulrich (1995) Planta 195, 440–449.
39. C.-H. Yang, L.-J. Chen, and Z. R. Sung (1995) Dev. Biol. 169, 421–435.
40. T. I. Baskin, A. Cork, R. E. Williamson, and J. R. Gorst (1995) Plant Physiol. 107, 233–242.
41. J.-D. Faure, P. Vittorioso, V. Santoni, V. Fraisier, E. Prinsen, I. Barlier, H. Van Onckelen, M. Caboche, and C. Bellini (1998) Development 125, 909–918.
42. T. Schmülling, S. Schäfer, and G. Romanov (1997) Physiol. Plant. 100, 505–519.
43. A. N. Binns (1994) Annu. Rev. Plant Physiol. Plant Mol. Biol. 45, 173–196.
44. P. J. Davies (1995) Plant Hormones: Physiology, Biochemistry and Molecular Biology, Kluwer, Dordrecht, The Netherlands.
الاكثر قراءة في مواضيع عامة في الاحياء الجزيئي
اخر الاخبار
اخبار العتبة العباسية المقدسة
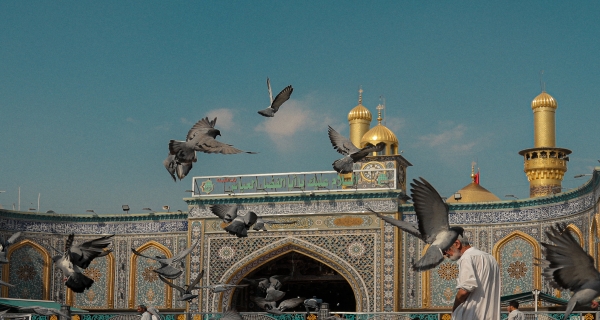
الآخبار الصحية
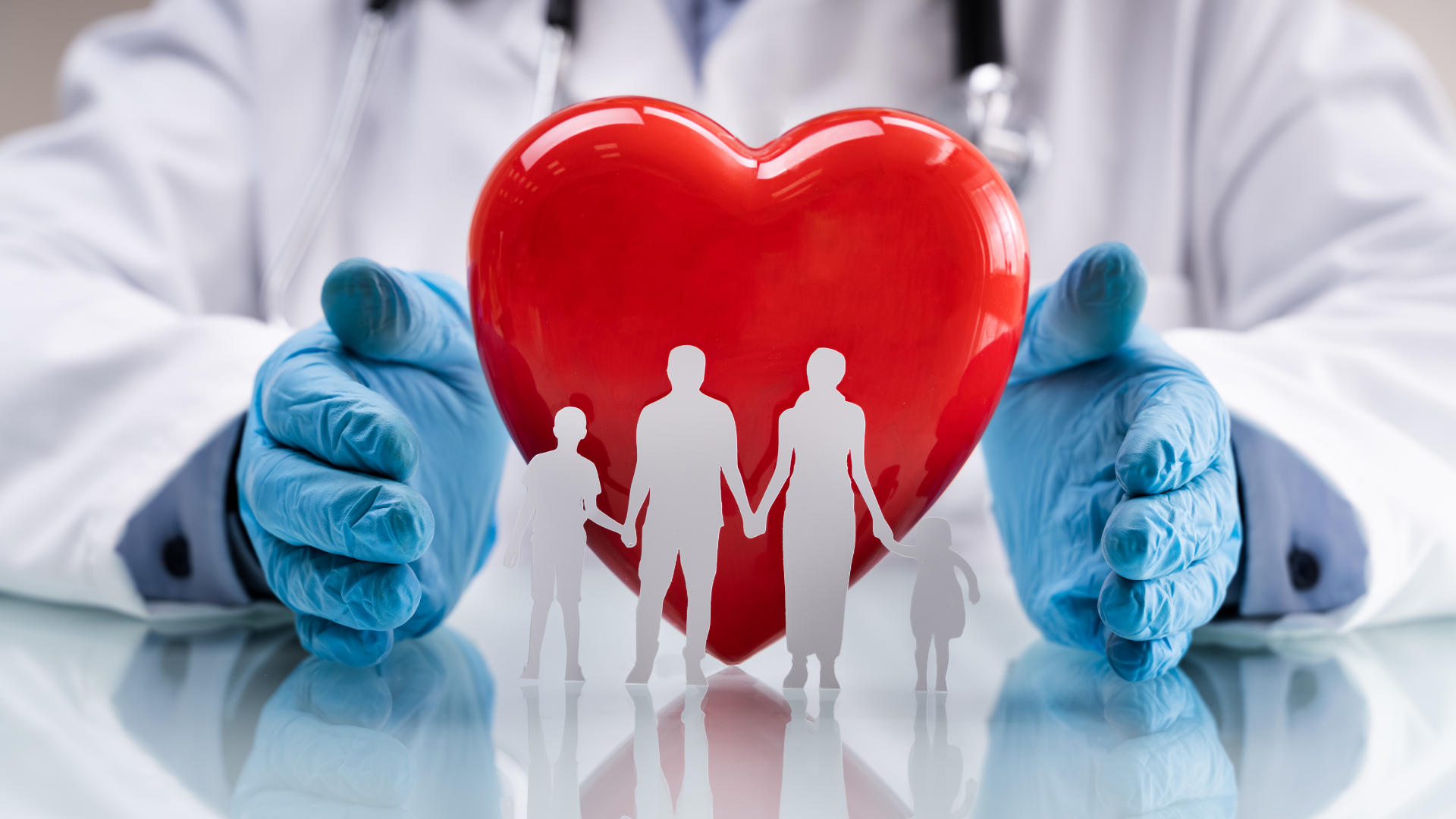