النبات
مواضيع عامة في علم النبات
الجذور - السيقان - الأوراق
النباتات الوعائية واللاوعائية
البذور (مغطاة البذور - عاريات البذور)
الطحالب
النباتات الطبية
الحيوان
مواضيع عامة في علم الحيوان
علم التشريح
التنوع الإحيائي
البايلوجيا الخلوية
الأحياء المجهرية
البكتيريا
الفطريات
الطفيليات
الفايروسات
علم الأمراض
الاورام
الامراض الوراثية
الامراض المناعية
الامراض المدارية
اضطرابات الدورة الدموية
مواضيع عامة في علم الامراض
الحشرات
التقانة الإحيائية
مواضيع عامة في التقانة الإحيائية
التقنية الحيوية المكروبية
التقنية الحيوية والميكروبات
الفعاليات الحيوية
وراثة الاحياء المجهرية
تصنيف الاحياء المجهرية
الاحياء المجهرية في الطبيعة
أيض الاجهاد
التقنية الحيوية والبيئة
التقنية الحيوية والطب
التقنية الحيوية والزراعة
التقنية الحيوية والصناعة
التقنية الحيوية والطاقة
البحار والطحالب الصغيرة
عزل البروتين
هندسة الجينات
التقنية الحياتية النانوية
مفاهيم التقنية الحيوية النانوية
التراكيب النانوية والمجاهر المستخدمة في رؤيتها
تصنيع وتخليق المواد النانوية
تطبيقات التقنية النانوية والحيوية النانوية
الرقائق والمتحسسات الحيوية
المصفوفات المجهرية وحاسوب الدنا
اللقاحات
البيئة والتلوث
علم الأجنة
اعضاء التكاثر وتشكل الاعراس
الاخصاب
التشطر
العصيبة وتشكل الجسيدات
تشكل اللواحق الجنينية
تكون المعيدة وظهور الطبقات الجنينية
مقدمة لعلم الاجنة
الأحياء الجزيئي
مواضيع عامة في الاحياء الجزيئي
علم وظائف الأعضاء
الغدد
مواضيع عامة في الغدد
الغدد الصم و هرموناتها
الجسم تحت السريري
الغدة النخامية
الغدة الكظرية
الغدة التناسلية
الغدة الدرقية والجار الدرقية
الغدة البنكرياسية
الغدة الصنوبرية
مواضيع عامة في علم وظائف الاعضاء
الخلية الحيوانية
الجهاز العصبي
أعضاء الحس
الجهاز العضلي
السوائل الجسمية
الجهاز الدوري والليمف
الجهاز التنفسي
الجهاز الهضمي
الجهاز البولي
المضادات الحيوية
مواضيع عامة في المضادات الحيوية
مضادات البكتيريا
مضادات الفطريات
مضادات الطفيليات
مضادات الفايروسات
علم الخلية
الوراثة
الأحياء العامة
المناعة
التحليلات المرضية
الكيمياء الحيوية
مواضيع متنوعة أخرى
الانزيمات
Cyclodextrins
المؤلف:
J. Szejtli
المصدر:
In Cyclodextrins and Their Inclusion Complexes
الجزء والصفحة:
30-12-2015
2884
Cyclodextrins
Cyclodextrins are cyclic oligosaccharides with a truncated cone shape and an axial void cavity (Fig. (1. The diameter and the volume of the cavity vary with the number of glucose units in the cyclodextrin ring (1). The most commonly used cyclodextrin is b-cyclodextrin, which has seven glucose units and a cavity with a diameter of 0.78 nm and a volume of approximately 35 nm3. Other natural cyclodextrins, such as a- and g-cyclodextrin, with six and eight glucose units and 0.57- and 0.95-nm cavity diameters, respectively, are also frequently used. The outer surface of the cyclodextrin molecule is hydrophilic, because the majority of the hydroxyl groups project outward, resulting in good water solubility. The internal cavity is relatively nonpolar, and it can encapsulate nonpolar solutes of appropriate dimensions, with binding occurring through various nonpolar interactions. Such binding is known as inclusion complexation.
Figure 1. Cyclodextrin structure.
The conformation of cyclodextrins in aqueous solution is believed to be that of the truncated cone of Figure 1. Molecules of hydrophobic compounds of appropriate size and shape penetrate into the cavity and are bound mainly through hydrophobic interactions, whose strengths depend on the efficiency of the contact. The edge of the torus of the larger circumference consists of secondary hydroxyl groups that are attached to chiral carbons (C2 and C3 of the glucose units). This structure results in variable binding affinities for different enantiomers, probably due to interactions of the chiral solute with the chiral entrance to the cavity (2). The primary hydroxyl groups of the glucose monomers make up the smaller edge of the cone. Chemical derivatization of natural cyclodextrins via modification of their hydroxyl groups is currently an area of very active research (3-6). Such modifications are yielding materials of varying complexation selectivities and physicochemical properties, such as improved solubility (7).
Hoffmann and Bock (8) have examined complex formation between different cyclodextrins and nucleotides. They found that adenosine, cytidine, guanosine, uridine, inositol, and deoxythymidine monophosphates (AMP, CMP, GMP, UMP, IMP, and dTMP) did not form a complex with a-cyclodextrin, but b-cyclodextrin readily bound AMP and IMP. It was concluded that that these six nucleotides are too bulky to fit into the cavity of a-cyclodextrin. When a complex is formed with b-cyclodextrin, the ribose and phosphate groups of the nucleotides exert a stabilizing effect by establishing hydrogen bonds with the outer rim of the cyclodextrin molecules. The position of the phosphate group is not important; increasing distance of the phosphate group from the base increased the stability of the complex. Larger oligonucleotides exhibited decreasing tendencies for complex formation, but the extent of complexation depended significantly on their base composition. Interestingly, polynucleotides with double- or triple-helical structures did not show any complexation with b-cyclodextrin (9). Transfer RNA, which comprises both helical and nonhelical structures, can interact with cyclodextrins, and these complexes have been used extensively for tRNA studies.
Cyclodextrins have also been used in labeling nucleic acid molecules in various biochemical analysis applications, especially in DNA sequencing. Cyclodextrin labels provide potentially high signal efficiency and versatility in label colors, while maintaining uniform chemical and physical properties. Cyclodextrin tracers are prepared by coupling the cyclodextrin to specific binding substances, such as nucleic acids, and forming inclusion complexes with the fluorophores. Then the DNA molecules are sequenced using cyclodextrin-labeled chain terminators. This method allows DNA sequencing with high sensitivity and high throughput (10).
Ikeda et al. (11) reported the use of anthryl(alkylamino)–cyclodextrin complexes as chemically switched DNA intercalators that were allosteric. On adding a ligand that is tightly bound in the cyclodextrin cavity, such as 1-adamantol, the host molecule releases the anthryl unit, which then leads to strong intercalation with the double-stranded DNA molecule. This principle could be extremely useful in nucleic acid reactions of medicinal and biotechnological importance, particularly in view of the established methods to modify cyclodextrins for specific interactions with a wide range of substances, for new drug delivery systems.
Cyclodextrins and their analogs can also be used as carriers to increase cellular uptake of phosphorothioate antisense oligonucleotides. Cellular uptake of phosphorothioate oligodeoxynucleotides in the presence of various cyclodextrin analogs was found to depend on the concentration and the time. In particular, 2-hydroxypropyl-b-cyclodextrin (HPbCD), 2-hydroxyethyl-b-cyclodextrin (HEbCD), and a mixture of various HPbCDs having different degree of substitution were observed to increase the uptake of phosphorothioate oligodeoxynucleotides two- to threefold in 48 h (12).
Cyclodextrins and derivatives are used to enhance the bioavailability of many water-insoluble pharmaceuticals (13). The solubilization results in fast and quantitative in vivo delivery for intravenous and intramuscular dosing. A decrease in irritation at the administered site can be observed (14). The solubility of hormones such as hydrocortisone was enhanced 72-fold using randomly methylated b-cyclodextrin (15). Sublingual administration of g-cyclodextrin complex of testosterone avoided rapid first-pass loss of the hormone and directed it effectively into the circulation; administration of the complex into the stomach resulted in much lower circulatory hormone levels (16).
The relatively good water solubility of the cyclodextrins makes these materials useful in chromatography and electrophoresis separation methods, such as in HPLC (high-performance liquid chromatography) and capillary zone electrophoresis (CZE) (17, 18). Buffer systems, even with organic modifiers, can be used to control the pH or modify other secondary equilibrium. Temperature also has a significant effect on selectivity in cyclodextrin-mediated separation systems.
References
1. J. Szejtli (1988) In Cyclodextrins and Their Inclusion Complexes, Cyclodextrin Technology, Kluywer, Dordrecht, The Netherlands.
2. A. Guttman et al. (1988) J. Chromatogr. 488, 41–53.
3. B. Sébille (1987) In Cyclodextrin Derivatives, Cyclodextrins and their Industrial Uses, D. Duchêne, ed., Editions de Santé, Paris, pp. 353–385.
4. K. Uekama (1985) Pharm. Intl. 6, 61–65.
5. J. Pitha et al. (1986) Intl. J. Pharm. 29, 73–82.
6. B. W. Müller and U. Brauns (1985) Intl. J. Pharm. 26, 77–88.
7. Y. Y. Rawjee, D. E. Stark, and Gy. Vigh (1993) J. Chromatogr. 635, 291–306.
8. L. Hoffmann and R. M. Bock (1970) Biochemistry 9, 3542–3550.
9. J. Szejtli, ed. (1982) Proceedings of the 1st International Symposium on Cyclodextrins, Reidel, Dordrecht, The Netherlands.
10. K. M. Kosak, PCT Intl. Appl. (WO 9102040, 2/21/1991), 69pp.
11. T. Ikeda, K. Yoshida, and H. J. Schneider (1995) J. Am. Chem. Soc. 117, 1453–1454.
12. Q. Zhao, J. Temsamani, and S. Agrawal (1995) Antisense Res. Devel. 5, 185–192.
13. R. A. Rajewski and V. Stella (1996) J. Pharm. Sci. 85, 1142–1169.
14.T. Loftsson and M. E. Brewster (1996) J. Pharm. Sci. 85, 1017–1025.
15. J. Szejtli (1994) Med. Res. Rev. 14, 353–386.
16.J. Pitha, E. J. Anaissie, and K. Uekama (1987) J. Pharm. Sci. 76, 788–790.
17. S. Ahuja (1991) In Chiral Separations by Liquid Chromatography, American Chemical Society, Washington, DC.
18. A. Guttman (1996) In Handbook of Capillary Electrophoresis, 2nd ed., J. P. Landers, ed., CRC Press, Boca Raton, pp. 75–100.
الاكثر قراءة في مواضيع عامة في الاحياء الجزيئي
اخر الاخبار
اخبار العتبة العباسية المقدسة
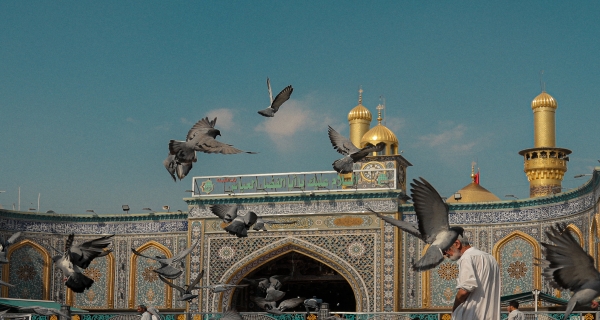
الآخبار الصحية
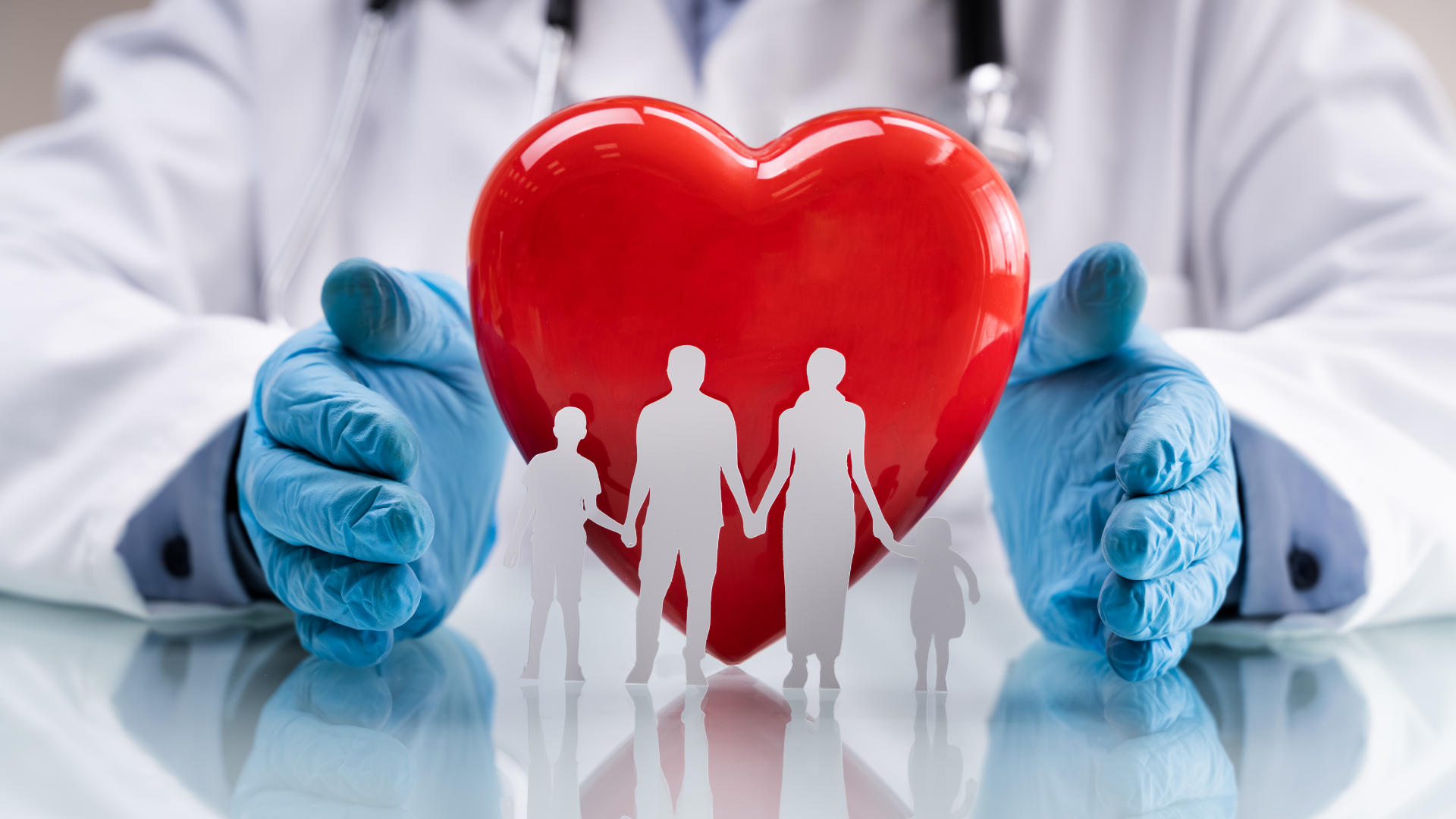