تاريخ الفيزياء
علماء الفيزياء
الفيزياء الكلاسيكية
الميكانيك
الديناميكا الحرارية
الكهربائية والمغناطيسية
الكهربائية
المغناطيسية
الكهرومغناطيسية
علم البصريات
تاريخ علم البصريات
الضوء
مواضيع عامة في علم البصريات
الصوت
الفيزياء الحديثة
النظرية النسبية
النظرية النسبية الخاصة
النظرية النسبية العامة
مواضيع عامة في النظرية النسبية
ميكانيكا الكم
الفيزياء الذرية
الفيزياء الجزيئية
الفيزياء النووية
مواضيع عامة في الفيزياء النووية
النشاط الاشعاعي
فيزياء الحالة الصلبة
الموصلات
أشباه الموصلات
العوازل
مواضيع عامة في الفيزياء الصلبة
فيزياء الجوامد
الليزر
أنواع الليزر
بعض تطبيقات الليزر
مواضيع عامة في الليزر
علم الفلك
تاريخ وعلماء علم الفلك
الثقوب السوداء
المجموعة الشمسية
الشمس
كوكب عطارد
كوكب الزهرة
كوكب الأرض
كوكب المريخ
كوكب المشتري
كوكب زحل
كوكب أورانوس
كوكب نبتون
كوكب بلوتو
القمر
كواكب ومواضيع اخرى
مواضيع عامة في علم الفلك
النجوم
البلازما
الألكترونيات
خواص المادة
الطاقة البديلة
الطاقة الشمسية
مواضيع عامة في الطاقة البديلة
المد والجزر
فيزياء الجسيمات
الفيزياء والعلوم الأخرى
الفيزياء الكيميائية
الفيزياء الرياضية
الفيزياء الحيوية
الفيزياء العامة
مواضيع عامة في الفيزياء
تجارب فيزيائية
مصطلحات وتعاريف فيزيائية
وحدات القياس الفيزيائية
طرائف الفيزياء
مواضيع اخرى
Entanglement Entropy
المؤلف:
Leonard Susskind And James Lindesay
المصدر:
AN INTRODUCTION TO BLACK HOLES, INFORMATION, AND THE STRING THEORY REVOLUTION
الجزء والصفحة:
16-12-2015
2031
Entanglement Entropy
In classical physics, the only reason for introducing a phase space probability is a lack of detailed knowledge of the state. In quantum mechanics, there is another reason, entanglement. Entanglement refers to quantum correlations between the system under investigation and a second system. More precisely, it involves separating a system into two or more subsystems.
Consider a composite system composed of 2 subsystems A and B. The subsystem A(B) is described by some complete set of commuting observables α (β).Let us assume that the composite system is in a pure state with wave function Ψ(α, β). Consider now the subsystems separately. All measurements performed on A(B) are describable in terms of a density matrix ρA (ρB).
(1.1)
The fact that a subsystem is described by a density matrix and not a pure state may not be due to any lack of knowledge of the state of the composite system. Even in the case of a pure state, the constituent subsystems are generally not described by pure states. The result is a “entanglement entropy” for the subsystems.
Let us consider some properties of the density matrix. For definiteness, consider ρA, but we could equally well focus on ρB.
1) The density matrix is Hermitian
(1.2)
2) The density matrix is positive semidefinite. This means its eigenvalues are all either positive or zero.
3) The density matrix is normalized to 1.
Tr ρ = 1 (1.3)
It follows that all the eigenvalues are between zero and one. If one of the eigenvalues of ρA is equal to 1, all the others must vanish. In this case the subsystem A is in a pure state. This only happens if the composite wave function factorizes
(1.4)
In this case B is also in a pure state.
4) The nonzero eigenvalues of ρA and ρB are equal if the composite system is in a pure state. To prove this, we start with the eigenvalue equation for ρA. Call φ the eigenvector of ρA. Then the eigenvalue condition is
We assume λ ≠ 0. Now we define a candidate eigenvector of ρB by
Then
Thus χ(β) is an eigenvector of ρB with eigenvalue λ.
From the equality of the non-vanishing eigenvalues of ρA and ρB an important property of entanglement entropy follows:
(1.5)
Thus we can just refer to the entanglement entropy as SE. The equality of SA and SB is only true if the combined state is pure. In that case, the entropy of the composite system vanishes
SA+B = 0
Evidently entropy is not additive in general.
Next, let us consider a large system Σ that is composed of many similar small subsystems σi. Let us suppose the subsystems weakly interact, and the entire system is in a pure state with total energy E. Each subsystem on the average will have energy ϵ.
It is a general property of most complex interacting systems that the density matrix of a small subsystem will be thermal
(1.6)
where Hi is the energy of the subsystem. The thermal density matrix maximizes the entropy for a given average energy ϵ. In general large subsystems or the entire system will not be thermal. In fact, we will assume that the entire system Σ is in a pure state with vanishing entropy.
The coarse grained or thermal entropy of the composite system is defined to be the sum of the entropies of the small subsystems
(1.7)
By definition it is additive. The coarse grained entropy is what we usually think of in the context of thermodynamics. It is not conserved. To see why, suppose we start with the subsystems in a product state with no corelations. The entropy of each subsystem Si as well as the entropy of the whole system Σ given by σΣ, and the coarse grained entropy of Σ all vanish.
Now the subsystems interact. The wave function develops correlations, meaning that it now fails to factorize. In this case, the subsystem entropies become nonzero
Si ≠ 0
and the coarse grained entropy also becomes nonzero
However, the “fine grained” entropy of Σ is exactly conserved and therefore remains zero.
Let us consider an arbitrary subsystem Σ1 of Σ which may consist of one, many, or all of the subsystems σi. Typically the fine grained entropy of Σ1 is defined as the entanglement entropy S(Σ1) of Σ1 with the remaining subsystem Σ−Σ1. This will always be less than the coarse grained entropy of Σ1
(1.8)
For example, as Σ1 approaches Σ, the fine grained entropy S(Σ1) will tend to zero.
Another concept that we can now make precise is the information in a subsystem. The information can be defined by
(1.9)
Often the coarse grained entropy is the thermal entropy of the system, so that the information is the difference between coarse grained and fine grained entropy.
Since typical small subsystems have a thermal density matrix, the information in a small subsystem vanishes. At the opposite extreme the information of the combined system Σ is just its total thermal entropy. It can be thought of as the hidden subtle correlations between subsystems that make the state of Σ pure.
How much information are in a moderately sized subsystem? One might think that the information smoothly varies from zero (for the σi) to SCoarseGrained (for Σ). However, this is not so. What actually happens is that for subsystems smaller than about 1/2 of the total system, the information is negligible.
Entropy and information are naturally measured in “bits”. A bit is the entropy of a two state system if nothing is known[2].The numerical value of a bit is log 2. Typically for subsystems less than half the size of Σ the information is smaller than 1 bit. The subsystem 1/2 Σ has about 1 bit of information. Thus for Σ1 < 1/2Σ
Next consider a subsystem with Σ1 > 1/2Σ.Ho w much information does it have? To compute it, we use two facts:
(1.10)
Thus
(1.11)
The coarse grained entropy of Σ−Σ1 will be of order (1 − f) SThermal(Σ), where f is the fraction of the total degrees of freedom contained in Σ1. Thus, for Σ1 < 1/2Σ the information in Σ1 is essentially zero. However forΣ1 > 1/2Σ we get the information to be
(1.12)
We will eventually be interested in the information emitted by a black hole when it evaporates. For now let's consider a conventional system which is described by known laws of physics. Consider a box with perfectly reflecting walls. Inside the box we have a bomb which can explode and fill the box with radiation. The box has a small hole that allows the thermal radiation to slowly leak out. The entire system Σ consists of the subsystem B that includes everything in the box. The subsystem A consists of everyting outside of the box, in this case, outgoing photons.
Initially the bomb is in its ground state, and B has vanishing entropy. When the bomb explodes, it fills the box with thermal radiation. The thermal, or coarse grained, entropy of the box increases, but its fine grained entropy does not. Furthermore, no photons have yet escaped, so S(A) = 0 at this time.
(1.13)
Next, photons slowly leak out. The result is that the interior and exterior of the box become entangled. The entanglement entropy, which is equal for A and B, begins to increase. The thermal entropy in the box decreases:
(1.14)
Eventually, all of the photons escape the box. The thermal or coarse grained entropy as well as the fine grained entropy in the box tends to zero. The box is in a pure state; its ground state.
Fig. 1.1. Top: Von Neumann entropy of Σ1 vs fraction f. Middle: coarse grained entropy of Σ1 vs fraction f. Bottom: information vs fraction of total degrees of freedom in Σ1.
At this time, the thermal or coarse grained entropy of the exterior radiation has increased to its final value. The second law of thermodynamics insures that SThermal(A) is larger than SThermal(B) just after the explosion. But the fine grained entropy of A must vanish, since the entanglement has gone to zero.
The actual entanglement entropy must be less than the thermal entropy of A or B. Thus a plot of the various entropies looks like Figure 1.2. Not that the point at which SThermal(A) = SThermal(B) defines the time at which the information in the outside radiation begins to grow. Before that point, a good deal of energy has escaped, but no information. Roughly the point where information appears outside of the box is the point where half of the final entropy of the photons has emerged.
It is useful to define this time at which information begins to emerge This time is called the information retention time. It is the amount of time that it takes to retrieve a single bit of information about the initial state of the box.
Thus we see how information conservation works for a conventional quantum system. The consequence of this principle is the final radiation field outside the box must be in a pure state. However, this does not mean that localized regions containing a small fraction of the photons cannot be extremely thermal. They typically carry negligible information.
The description of the evolution of the various kinds of entropy follow from very general principles. Thus we regard the conservation of information in black hole evaporation as a fundamental law of nature. Note that it applies to observations made from outside the black hole.
الاكثر قراءة في الثقوب السوداء
اخر الاخبار
اخبار العتبة العباسية المقدسة
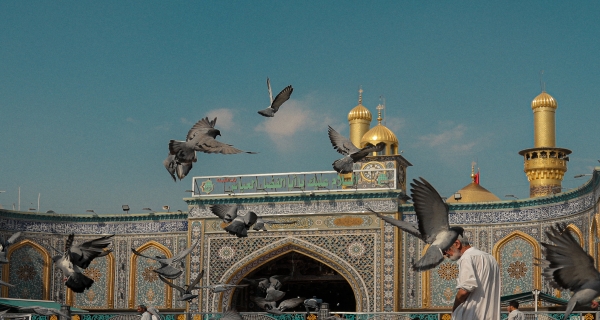
الآخبار الصحية
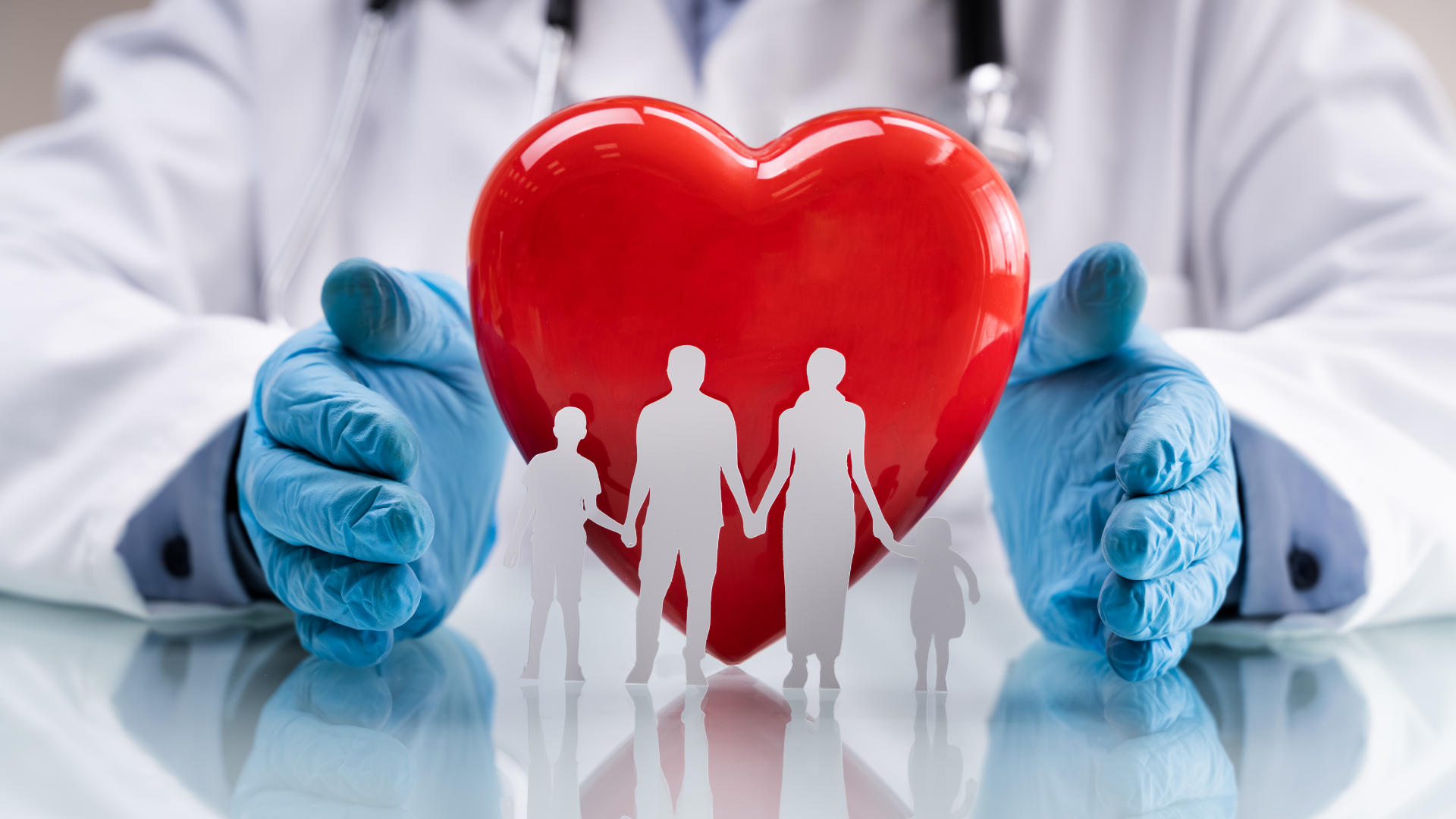