النبات
مواضيع عامة في علم النبات
الجذور - السيقان - الأوراق
النباتات الوعائية واللاوعائية
البذور (مغطاة البذور - عاريات البذور)
الطحالب
النباتات الطبية
الحيوان
مواضيع عامة في علم الحيوان
علم التشريح
التنوع الإحيائي
البايلوجيا الخلوية
الأحياء المجهرية
البكتيريا
الفطريات
الطفيليات
الفايروسات
علم الأمراض
الاورام
الامراض الوراثية
الامراض المناعية
الامراض المدارية
اضطرابات الدورة الدموية
مواضيع عامة في علم الامراض
الحشرات
التقانة الإحيائية
مواضيع عامة في التقانة الإحيائية
التقنية الحيوية المكروبية
التقنية الحيوية والميكروبات
الفعاليات الحيوية
وراثة الاحياء المجهرية
تصنيف الاحياء المجهرية
الاحياء المجهرية في الطبيعة
أيض الاجهاد
التقنية الحيوية والبيئة
التقنية الحيوية والطب
التقنية الحيوية والزراعة
التقنية الحيوية والصناعة
التقنية الحيوية والطاقة
البحار والطحالب الصغيرة
عزل البروتين
هندسة الجينات
التقنية الحياتية النانوية
مفاهيم التقنية الحيوية النانوية
التراكيب النانوية والمجاهر المستخدمة في رؤيتها
تصنيع وتخليق المواد النانوية
تطبيقات التقنية النانوية والحيوية النانوية
الرقائق والمتحسسات الحيوية
المصفوفات المجهرية وحاسوب الدنا
اللقاحات
البيئة والتلوث
علم الأجنة
اعضاء التكاثر وتشكل الاعراس
الاخصاب
التشطر
العصيبة وتشكل الجسيدات
تشكل اللواحق الجنينية
تكون المعيدة وظهور الطبقات الجنينية
مقدمة لعلم الاجنة
الأحياء الجزيئي
مواضيع عامة في الاحياء الجزيئي
علم وظائف الأعضاء
الغدد
مواضيع عامة في الغدد
الغدد الصم و هرموناتها
الجسم تحت السريري
الغدة النخامية
الغدة الكظرية
الغدة التناسلية
الغدة الدرقية والجار الدرقية
الغدة البنكرياسية
الغدة الصنوبرية
مواضيع عامة في علم وظائف الاعضاء
الخلية الحيوانية
الجهاز العصبي
أعضاء الحس
الجهاز العضلي
السوائل الجسمية
الجهاز الدوري والليمف
الجهاز التنفسي
الجهاز الهضمي
الجهاز البولي
المضادات الحيوية
مواضيع عامة في المضادات الحيوية
مضادات البكتيريا
مضادات الفطريات
مضادات الطفيليات
مضادات الفايروسات
علم الخلية
الوراثة
الأحياء العامة
المناعة
التحليلات المرضية
الكيمياء الحيوية
مواضيع متنوعة أخرى
الانزيمات
Bacteriocins
المؤلف:
H. G. Sahl
المصدر:
In Antimicrobial Peptides
الجزء والصفحة:
10-12-2015
6569
Bacteriocins
Broadly defined, bacteriocins are substances produced by one bacterium that adversely affect another. Most of them are peptide antibiotics that are synthesized on ribosomes, as in normal protein biosynthesis. Others, such as bacitracin and gramicidin, are synthesized in bacteria by multienzyme complexes or sequential enzyme reactions (1). In a strict sense, bacteriocin is an abbreviation of bacterial toxin (with antibacterial activity). Many bacterial toxins act against eukaryotic cells and are not called bacteriocins. Some of them, however, like diphtheria, tetanus or cholera toxins, resemble bacteriocins, such as colicins or pyocins, in having domain structures that convey the binding and toxic activities specific to each molecule (2, 3). The current basis for allocating a name to the agent responsible for bacteriocinlike activity produced by Gram-positive or -negative bacteria is to adopt some derivation of either the genus or species name of the producer strain, together with an alphabetical and/or numerical code designation specifying that strain. To avoid confusion, bacteriocins that have the same amino acid sequence, irrespective of the species of origin, should have the same name, that first published. There is a wide variety of locations of the genetic determinants of bacteriocins. They can be encoded by conjugative or nonconjugative plasmids, by conjugative transposons, or be encoded on the chromosome (4).
Because of the different cell-wall structures of gram-positive or -negative bacteria, their bacteriocins have evolved differently in size and specificity. In gram-negative bacteria, the outer membrane necessitates receptor-mediated antagonistic activities, and very specific proteins are produced with domains for receptor binding, translocation, and activity. In contrast, gram-positive bacteria possess a multilayered peptidoglycan wall without an outer membrane. This favors peptides of small size that penetrate the murein network without receptor binding and specific translocation. Consequently, bacteriocins produced by gram-positive bacteria have a broad host range and are sometimes active on taxonomically unrelated genera. Thus, many of the bacteriocinlike agents produced by gram-positive bacteria kill species other than those likely to have the same ecological niche. Production of antibiotic peptides is the rule in gram-positive bacteria. Proteins the size of colicins are hardly ever encountered. In general the structures of these peptides must be stabilized by posttranslational modifications.
Presently there is a burgeoning interest in bacteriocins because of the possible applications in the food industry (particularly lactic acid bacteria) and as a strategy for preventing certain infectious diseases. This review gives the general features of the main classes of bacteriocins and emphasizes the common features that are beginning to emerge.
An organism that produces a peptide antibiotic must be able to (1) synthesize the antibiotic, (2(export the antibiotic into the extracellular medium, (3) protect itself from the action of the antibiotic, and (4) interact with the sensitive cell and interfere with its growth or survival. This interaction may or may not require entry of the antibiotic into the cell. Such entry is characteristic of some of the better-characterized ribosomally synthesized low molecular weight bacteriocins. For a detailed description of these bacteriocins, the reader is referred to the Suggestions for further reading.
1. Classes of Bacteriocins
A list of representative examples of lantibiotic and non-lantibiotic bacteriocins, together with their organisms of origin and mode of action is presented in Table 1. Four distinct classes of bacteriocins from lactic acid bacteria have been defined (5).
Table 1. Representative Examples of Lanthionine-Containing Bacteriocins
Class I
The lantibiotics (lanthionine-containing peptides) are small antibiotic peptides that are distingue content of dehydro and thioether amino acids (lanthionine and 3-methyl-lanthionine) (6). Two their distinctive ring structure. Type A comprises screw-shaped, amphipathic molecules that ha two to seven net positive charges. Type B consists of more globular molecules that have molecules charge or a net negative charge. The lantibiotics include a growing list of modified peptides that bacilli, lactococci, lactobacilli, staphylococci, streptococci, and Streptomyces (Fig. 1). The function twofold. First, these small peptide molecules are less likely to achieve stable conformations by they are generally extremely heat stable. Unusual cross-links like the thioether bridge from lant retain their proper folded structure despite their small size (7). Secondly, unusual sidechains, su repertoire of chemical reactivity that is be important for the biological activity of these peptide
Figure 1. Primary structure of representative lantibiotics. Abbreviations used: dhA, didehydroalanine; dhB, dide ß-methyl lanthionine; Ala-NH-Lys, lysinoalanine; Asp-OH, hydroxyaspartic acid.
Class II
These bacteriocins are small (<10 kDa), relatively heat stable, non-lanthionine-containing peptide been subdivided into Class IIa, Listeria-active peptides with the N-terminal consensus sequence IIb, poration complexes requiring two different peptides for activity; Class IIc, thiol-activated p for activity. Unmodified peptide antibiotics (not containing lanthionine) share several character propeptides that has an N-terminal extension (leader peptide). Leader peptides are amphiphilic hydrophobic region of the sec-dependent exported proteins (see protein targeting). The leader particularly at the proteolytic processing site, where two glycine residues are found at position (4, 9) .The processed, active bacteriocins contain a substantial amount of hydrophobic amino Accordingly, the plasma membrane is the target of most of these bacteriocins. Flanking regions gene of non-lantibiotic peptides contain additional open reading frames for which a function demonstrated or postulated on the basis of sequence homologies. Such genes code for immunity proteins consisting of a histidine kinase and the respective regulator, and for proteins with sign family. Some of these genes have an operon-like organization.
Class III
These bacteriocins are large (>30 kDa) heat-labile proteins that include many bacteriolytic extra muramidases) that mimic the physiological action of bacteriocins.
Class IV
These are complex bacteriocins that contain essential lipid or carbohydrate moieties an in addition purified antibacterial component is still necessary to confirm that the presence of the additional biological activity of these molecules, thereby justifying the establishment of this class IV.
1.1. Microcins
An additional class of bacteriocins comprises a family of antibiotic substances called “microcins” produced by diverse members of the Enterobacteriacae (10). They are distinguished from the majority of colicins by much lower molecular weight (<10 kDa) and because their synthesis is not induced by conditions that lead to induction of the SOS repair pathway. Instead, they are synthesized during the stationary phase, similar to that observed with most conventional antibiotics (11). The microcins were operationally defined as substances produced by gram-negative bacteria that pass through a cellophane membrane and inhibit the growth of an indicator Escherichia coli strain (12). Several substances known to be ribosomally synthesized fall within this group. The best known of these antibiotics peptides are microcin C7, microcin B17, and colicin V. Microcin C7 is a heptapeptide containing modifications at both the N- and C-termini that block protein synthesis in vivo and in vitro (13). Microcin B17 (Mcc B17) is a 43-residue peptide containing posttranslational modifications at the peptide backbone including serine, cysteine, and glycine residues, that result in thiazole and oxazole rings (14). Mcc B17 has much in common with lantibiotics because it is derived from a precursor peptide that is posttranslationally modified; 26 out of the 43 residues are glycine. Mcc B17 inhibits DNA replication and induces the SOS response (15). The target of this antibiotic is a DNA gyrase (16). Colicin V (ColV) was first described in 1925 in the first report of an antibiotic substance produced by E. coli (17). It has a molecular weight of only 6000. The structure of mature colicin V is not totally known, even regarding side-chain modifications or N-terminal processing. Colicin V kills sensitive cells by disrupting their membrane potential (18).
2. Biosynthesis and Posttranslational Modifications
Generally the low molecular weight bacteriocins of gram-positive bacteria are first formed in an inactive precursor form that has a leader peptide. Following a variety of posttranslational modification reactions, the C-terminal propeptide domain is cleaved from the N-terminal leader sequence to yield the mature antimicrobial molecule. These prepeptides range from 18 to 30 amino acid residues and are not highly homologous except near the cleavage site. The prepeptides of all non-lanthionine-containing bacteriocins characterized thus far have two Gly residues at positions –2 and –1 relative to the processing site and b-turn promoting residues near the cleavage site. Possible functions of the prepeptide include (1) stabilizing the propeptide during translation; (2) keeping the bacteriocin inactive, particularly after completion of modifications and thus helping to protect the producing strain; (3) allowing recognition of the ABC transporter system; (4) directing the precursor through a specific recognition motif toward biosynthetic enzymes (modification enzymes); (5(interacting with the propeptide region to stabilize a conformation that is essential for correct modification and thioether formation. There is evidence that the modifications of lantibiotics are made at the prepeptide stage. Cross-similarities between leaders of the modified and unmodified bacteriocins indicate that the overall features of leader peptides are important during regulation, synthesis, and generation of the immunity of peptide bacteriocins, regardless of whether or not they are modified.
Table 2. Non-Lanthionine-Containing Bacteriocins
The primary amino acid sequences of the propeptide components of many of the low molecular weight bacteriocins produced by gram-positive bacteria are known now. One important feature of the bacteriocins of gram-positive bacteria is their cysteine content. Those in which one or more cysteine residues are linked to dehydrated serine and threonine residues to form the thioether-linked amino acids lanthionine and methyl-lanthionine are called lantibiotics as previously mentioned (Fig. 1). Alternatively, bacteriocins in which pairs of cysteine residues undergo modification to form disulfide bonds are called cystibiotics (cysteine-containing antibiotics). A third subgroup of the bacteriocins, of which lactococin B is an example, are designated thiolbiotics, because they contain only a single cysteine residue that must be present in the reduced thiol form to be active (19). At neutral pH, many of the low molecular weight bacteriocins are cationic. This is a unifying feature of lantibiotic and non-lanthionine containing bacteriocins and may have some significance for their activity.
Little is known about the posttranslational modification reactions and the biosynthetic enzymes involved in the maturation of lantibiotics. The lantibiotic B and C genes (designated LanB and LanC, as stipulated by the 1st and 2nd International Workshops on Lantibiotics) are probably involved in the maturation pathways, because mutation studies indicate that the gene products are essential for producing functional lantibiotics. The products of these genes, designated LanB and LanC, but also known as NisB and NisC, respectively, may be involved in the dehydration and thioether bond formation. Limited similarity was reported between LanB and E. coli IlvA, a threonine dehydratase, thus suggesting a similar function (20). This leads to the hypothesis that LanC and other similar proteins are involved in the enzyme-catalyzed formation of thioether bonds from the dehydrated residues and cysteine. Another modification enzyme that has been identified is EpiD, which is encoded by the epidermin gene cluster located on the 54-kb plasmid pTu32 (21). EpiD has no homologue in other lantibiotic gene clusters, and it catalyzes the biosynthesis of the C-terminal aminovinylcysteine residue of epidermin (22).
3. Extracellular and Maturational Release
After biosynthesis, class I and II bacteriocins are released to the extracellular medium. For each bacteriocin there is a relatively specific membrane protein system whose function is to translocate a precursor form of the antibiotic across the cytoplasmic membrane to the outside of the cell. The secretion of several peptide bacteriocins is mediated by dedicated transmembrane translocators belonging to the ATP-binding cassette (ABC) transporter superfamily (23-26). The transporters are encoded in the same operons as the bacteriocin structural gene or on a neighboring operon. The C-terminal ATP-binding domain and the N-terminal hydrophobic integral membrane domain are expressed as a single polypeptide or as separate polypeptides. Recently, strong evidence has been reported that, very likely, all precursor peptides of the lantibiotic and non-lantibiotic bacteriocins that have leader peptides of the double glycine type (see above) are processed by their dedicated ABC-transporters, concomitant with export (27). It has also been proposed that the N-terminal domains of bacteriocin transporters are essential for initial recognition and subsequent proteolytic processing. These new proteolytic enzymes are thiol proteinases. In fact, genes for potential ABC transporters have been found in all the known lantibiotic gene clusters. The lantibiotic transporters range in size from 535 to 714 amino acid residues and generally have the ATP-binding domain and the membrane-spanning domain in the same single protein. Sometimes a second ABC transporter system has been identified, which is not essential for production but participates in producer self-protection, thus providing some type of additional immunity (28). Although the leader sequence of the double-glycine type may be cleaved concomitant with export by the ABC-transporter itself, this is not the general case.
The ABC transporters have thiol proteinase activity, and putative proteinase genes (designated P) have been described for the leader peptides of other lantibiotics. These gene products share similarities with subtilisin-like serine proteinases. For example, the NisP gene encodes an extracellular serine proteinase with a C-terminal extension anchoring it on the outer side of the cytoplasmic membrane (29). With nisin, it has been shown that cleavage of the leader peptide is not a prerequisite for export by the ABC-transporter, and this conclusion may be extended to other peptides with antimicrobial activity (30).
A detailed maturational pathway has been proposed for nisin (31). First the inducing signal (which could be nisin itself) activates the transcription at the nisA promoter (which has features also found in other positively regulated promoter sequences) via a two-component response-regulator system (32 .This results in the production of pre-nisin containing free cysteines and no dehydrated residues. The pre-nisin is directed, presumably by virtue of the leader peptide, to a membrane-located complex containing the modifying enzymes LanB (probably involved in dehydration) and LanC (probably involved in thioether bonds, as previously mentioned). At this stage, the leader helps to maintain the peptide in an inactive form. Subsequently, the precursor nisin is translocated via the ABC exporter NisT at the expense of ATP hydrolysis. Finally, precursor nisin is activated by proteolytic cleavage by the extracellular protease NisP attached to the outside of the cell envelope. The role of the leader is still not fully understood, apart from its function in producing an inactive conformation. In particular, it is not known how it functions in targeting the pre-lantibiotic to the maturation and export proteins, what its fate is after cleavage, and how it contributes to processes, such as self protection, after it has been cleaved off.
This proposed sequence of events may also apply to other lantibiotics. However, additional intracellular conversions are required in specific modification reactions, such as those involving the N- and C-termini of epidermin (33), lantibiotic Pep5, epilamin K7 (34, 35), and lactocin S (36). In these cases it is likely that the leader peptide cleavage occurs intracellularly.
4. Modes of Action
The various structural properties of the subgroups of bacteriocins are reflected in their three different modes of action. The primary activity of Class I type A lantibiotics is based on forming voltage-dependent, short-lived pores in the cytoplasmic membrane (37, 38). The peptides rapidly induce leakage of ions and small metabolites from bacterial cells and a collapse of the electrochemical proton gradient, leading to cessation of biosynthetic processes and eventually to cell death. Type A lantibiotics require a membrane potential of between 50 and 100 mV for pore formation, depending on the individual peptide. It is assumed that pores are formed by a transiently associated peptide oligomer in a transmembrane orientation (barrel stave model), as suggested for alamethicin. The susceptibility toward a particular peptide of different bacterial species, or even of strains within one species, varies much more than one would expect on the basis of the pore-formation model. In vivo pore formation or pore stability may be positively or negatively influenced by such factors as phospholipid composition of the membrane, interactions of the peptides with integral membrane components, or the presence of surface layers. The models may need to be refined, and pore formation may depend on local perturbation of the bilayer (39). In addition, the peptides could exert secondary effects that contribute to bactericidal activity, such as autolysis of cells by activating cell-wall hydrolyzing enzymes (40).
The antibacterial effects of class I type B lantibiotics are rather weak. They bind to the head groups of phospholipids, preferentially to phosphoethanolamine, which may cause membrane permeabilization and allow the release of cations and other small solutes. Duramycin also induces the formation of complex pores (41). Mersacidin and actagardine are distinguished from other type B lantibiotics by their mode of action. Both interfere with cell-wall biosynthesis in gram-positive bacteria, which may eventually offer new possibilities in antimicrobial therapy (42).
Generally, the bactericidal action of class II bacteriocin against sensitive cells is produced principally by destabilizing membrane function, such as energy transduction, rather than disrupting the structural integrity of the membrane. This effect results from the energy-independent dissipation of the proton motive force and loss of the permeability barrier of the cytoplasmic membrane. It contrasts with the energy-dependent bactericidal action of the lantibiotics. In addition, before pores are formed, all of the non-lanthionine containing bacteriocins interact with membrane-associated receptor proteins, in contrast to class I lantibiotic bacteriocins.
5. Immunity of Bacteriocin-Producing cells
One of the definitive features of bacteriocin-producing cells is their ability to resist the action of their own inhibitory substances through a specific immunity mechanism. Such a mechanism is based on dedicated peptides or proteins called immunity proteins, which specifically antagonize the bacteriocin. For nisin and subtilisin, their antagonist proteins of 245 and 165 amino acid residues, respectively, display the features of bacterial lipoproteins and are similar in amino acid sequence. Despite the high degree of similarity of these lantibiotics, there is no cross-immunity between producing cells. In other cases, as with pepS, the immunity is much more closely related to the immunity systems of unmodified bacteriocins, which are short, have only 69 residues, and have a hydrophobic N-terminal segment and a strongly hydrophilic C-terminal part.
Currently, there are no clues to the mechanism of the immunity phenomenon. The proposed location of the peptides outside the cell and the observation that the cytoplasmic membrane of immune clones is not depolarized by externally added bacteriocin suggest that the bacteriocins are directly antagonized by the immunity peptide, like colicin A, for example (43).
References
1.H. Kleinhauf and H. von Döhren (1990) Eur. J. Biochem. 192, 1–15.
2.M. Kageyama, M. Kobayashi, Y. Sano, and H. Masaki (1996) J. Bacteriol. 178, 103–110.
3.H. Bénédetti and V. Géli (1996) In Handbook Biological Physics (W. N. Konings, H. R. Kaback, and J. S. Lolkema eds.), Elsevier, Amsterdam, The Netherlands, Vol. 2, pp. 665–691.
4.H. G. Sahl (1994) In Antimicrobial Peptides (Ciba Foundation Symposium 186), Wiley, Chichester, pp. 27–53.
5.T. R. Klaenhammer (1988) Biochimie 70, 337–349.
6.G. Jung (1991) Angew. Chem. Int. Ed. Engl. 30, 1051–1068.
7.E. Gross and J. L. Morell (1967) J. Amer. Chem. Soc. 53, 2791–2792.
8.E. Gross and J. L. Morell (1971) J. Amer. Chem. Soc. 93, 4634–4635.
9زW. M. de Vos, O. P. Knipers, J. R. van der Meer, and R. J. Siezen (1995) Mol. Microbiol. 17, 427-337 .
10. F. Baquero and F. Moreno (1984) FEMS Microbiol. Lett. 23, 117–124.
11.J. F. Martin and A. L. Demain (1980) Microbiol. Rev. 44, 230–251.
12.C. Asensio, C. Perez-Diaz, M. C. Martinez, and F. Baquero (1976) Biochem. Biophys. Res. Commun. 69, 7–14.
13.R. Kolter and F. Moreno (1992) Ann. Rev. Microbiol. 46, 141–163.
14.A. Bayer, S. Freund, C. Nicholson, and C. Jung (1993) Angew. Chem. Int. Ed. Engl. 32, 1336–1339.
15.M. Herrero and F. Moreno (1986) J. Gen. Microbiol. 132, 393–402.
16.J. L. Vizan, C. Hernandez-Chico, I. del Castillo, and F. Moreno (1991) EMBO J. 10, 467–476.
17.A. Gratia (1925) C.R. Soc. Biol. 93, 1040–1041.
18.C. Yang and J. Konisky (1984) J. Bacteriol. 158, 757–759.
19.G. Bierbaum and H. Sahl (1991) In Nisin and Novel Antibiotics (G. Jung and H. G. Sahl, eds.(, Escom Publishers, Leiden, The Netherlands, pp. 386–396.
20.Z. Gutowski-Eckel, C. Klein, K. Siegers, K. Bohm, M. Hammelmann, and K. D. Entian (1994) Appl. Environ. Microbiol. 60, 1–11.
21.N. Schnell, G. Engelke, R. Augustin, F. Rosenstein, F. Götz, and K. D. Entian (1991) In Nisin and Novel Antibiotics (G. Jung and H. G. Sahl, eds.), Escom Publishers, Leiden, The Netherlands, pp. 269–276.
22.T. Kupke, S. Stefanovic, H. G. Sahl, and F. Götz (1992) J. Bacteriol. 174, 5354–5361.
23.L. Gilson, H. K. Mahanty, and R. Kolter (1990) EMBO J. 9, 3875–3884.
24.M. S. Gilmore, R. A. Segarra, and M. C. Booth (1990) Infect. Immunol. 58, 3914–3923.
25.J. D. Marugg, C. F. Gonzalez, B. S. Kunka, A. M. Ledeboer, M. J. Pucci, M. Y. Toonen, S. A. Walker, L. C. Zoetmulder, and P. A. Vandebergh (1992) Appl. Environ. Microbiol. 58, 2360–2367.
26.G. W. Stoddard, J. P. Petzel, M. J. van Belkum, J. Kok, and L. L. McKay (1992) Appl. Environ. Microbiol. 58, 1952–1961.
27.L. S. Havarstein, H. Holo, and I. F. Nes (1994) Microbiol. 140, 2393–2389.
28.K. Venema, G. Venema, and J. Kok (1995) Trends Biochem. Sci. 3, 299–304.
29.J. R. van der Meer, J. Polman, M. M. Beerthuyzen, R. J. Siezen, O. P. Kuipers, and W. M. de Vos (1993) J. Bacteriol. 175, 2578–2588.
30.J. R. van der Meer, H. S. Rollema, R. J. Siezen, M. M. Bethuyzen, O. P. Kuipers, and W. M. de Vos (1994) J. Biol. Chem. 269, 3555–3562.
31.W. M. de Vos, O. P. Kuipers, J. R. van der Meeer, and R. Siezen (1995) Mol. MIcrobiol. 17, 427-437.
32.W. M. de Vos and G. F. Simons (1994) In Genetics and Biotechnology of Lactic Acid Bacteria ) M. J. Gasson and W. M. de Vos, eds.), Backie Academic, Glasgow, pp. 52–105.
33.T. Kupke, C. Kempter, V. Gnan, G. Jung, and F. Götz (1994) J. Biol. Chem. 269, 5653–5659.
34.M. Reis, M. Eschbach-Bludau, M. Iglesias-Wind, T. Kupke, and H. G. Sahl (1994) Appl. Environ. Microbiol. 60, 2876–2883.
35.M. van de Kamp, H. W. van den Hooven, R. N. Konings, C. W. Hilbers, C. W. van de Ven, G. Bierbaum, H. G. Sahl, O. P. Kuipers, R. J. Seizen, and W. M. de Vos (1995) Eur. J. Biochem. 230, 587-600.
36.M. Skangen, J. Nissen-Meyer, G. Jung, S. Stefanovic, K. Sletten, C. I. Mortreveldt-Abilgaard, and I. F. Nes (1994) J. Biol. Chem. 269, 27183–27185.
37.H. G. Sahl (1991) In Nisin and novel lantibiotics Proceedings of the First International Workshop on Lantibiotics (G. Jung and H. G. Sahl, eds.), Leiden, Escom Publishers, pp.347–359.
38.R. Benz, G. Jung, and H. G. Sahl (1991) In Nisin and novel lantibiotics Proceeding of the First International Workshop on Lantibiotics (G. Jung and H. G. Sahl, eds.), Leiden, Escom Publishers, pp. 359–372.
39.A. J. Driessen, H. W. van den Hooven, W. Kuiper, M. van de Kamp, H. G. Sahl, R. N. Konings, and W. Konings (1995) Biochemistry 34, 1606–1614.
40.G. Bierbaum and H. G. Sahl (1987) J. Bacteriol. 169, 5452–5458.
41.T. Sheth, R. M. Henderson, S. B. Hlady, and W. A. Cuthbert (1992) Biochim. Biophys. Acta 1107, 179–185.
42.S. Chatterjee, D. Chatterjee, K. Jani, H. Blumbach, B. Ganguli, N. Klesel, M. Limbert, and G. Seibert (1992) J. Antibiot. 45, 839–845.
43.D. Espesset, D. Duché, D. Baty, and V. Géli (1996) EMBO J. 15, 2356–2364.
44.R. Kellner, G. Jung, and H. G. Sahl (1991) In Nisin and Novel Lantibiotics (G. Jung and H. G. Sahl, eds.), Escom Publishers, Leiden, The Netherlands, pp. 141–158.
45.E. Gross, H. H. Kitz, and E. Nebelin (1973) Hoppe-Seyler''s Z. Physiol. Chem. 354, 810–812.
46.M. van de Kamp, L. Horstink, H. W. van den Hooven, R. N. Konings, C. W. Hilbers, A. Frey, H. G. Sahl, J. W. Metzger, and F. J. van de Ven (1995) Eur. J. Biochem. 227, 757–771.
47.H. Allgaier, G. Jung, R. Werner, U. Schneider, and H. Zähner (1986) Eur. J. Biochem. 160, 9–22.
. 48R. Kellner, G. Jung, T. Hörner, H. Zähner, N. Schnell, K. Entian, and F. Götz (1988) Eur. J. Biochem. 177, 53–59.
49.J. C. Piard, C. Delorme, M. Novel, M. Desmageaud, and G. Novel (1993) FEMS Microbiol. Lett. 112, 313–318.
50.R. W. Jack, A. Carne, J. Metzger, S. Stefanovic, H. G. Sahl, G. Jung, and J. R. Tagg (1994) Eur. J. Biochem. 220, 455–462.
51.K. F. Ross, C. Ronson, and J. R. Tagg (1993) Appl. Environ. Microbiol. 59, 2014–2021.
52.J. Novak, P. W. Canfield, and E. J. Miller (1994) J. Bacteriol. 176, 4316–4320.
53.G. Stoffels, J. Niessen-Meyer, A. Gudmundsdottir, K. Sletten, H. Halo, and I. F. Nes (1992(Appl. Environ. Microbiol. 58, 1417–1422.
54.M. S. Gilmore, R. A. Serraga, M. C. Booth, C. P. Bogie, L. R. Hall, and D. B. Clewell (1994) J. Bacteriol. 176, 7335–7344.
55.A. Fredenhagen, F. Märki, G. Fendich, W. Märki, J. Gruner, J. van Oostrum, F. Raschdorf, and H. H. Peter (1991) In Nisin and Novel Lantibiotics (C. Jung and H. G. Sahl eds), Escom Publishers, Leiden, The Netherlands, pp. 131–140.
56.H. Kogler, H. Bauch, H. W. Fehlhaber, C. Griesinger, W. Schubert, and V. Teetz (1991) In Nisin and Novel Lantibiotics (G. Jung and H. G. Sahl, eds.), Escom Publishers, Leiden, The Netherlands, pp. 159–170.
57.N. Zimmermann, N. S. Freud, A. Fredenhagen, and G. Jung (1993) Eur. J. Biochem. 216, 419–428 .
58.M. L. Chikindas, M. J. Garcia-Garcera, A. J. Driessen, A. M. Ledeboer, J. Niessen-Meyer, I. F. Nes, T. Abee, W. N. Konings, and G. Venema (1993) Microbiology 59, 3577–3584.
59.A. Maftah, T. Renault, C. Viguoles, Y. Hechard, P. Bressolier, M. Ratineaud, Y. Cenatiempo, and R. Julian (1993) J. Bacteriol. 175, 3232–3235.
60.R. W. Woboro, T. Henkel, M. Sailer, K. L. Roy, J. C. Vederas, and M. E. Skiles (1994(Microbiology 140, 517–526.
61.A. Holck, L. Axelson, S. S. E. Birkeland, T. Aukrust, and H. Bloom (1992) J. Gen. Microbiol 138,. 2715–2720
62.P. Muriana and T. R. Klaenhammer (1991) J. Bacteriol. 173, 1779–1788.
63. H. Holo, O. Niessen, and I. F. Ness (1991) J. Bacteriol. 173, 3879–3887.
الاكثر قراءة في مواضيع عامة في الاحياء الجزيئي
اخر الاخبار
اخبار العتبة العباسية المقدسة
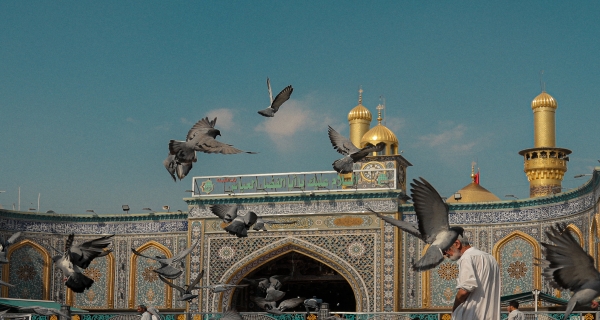
الآخبار الصحية
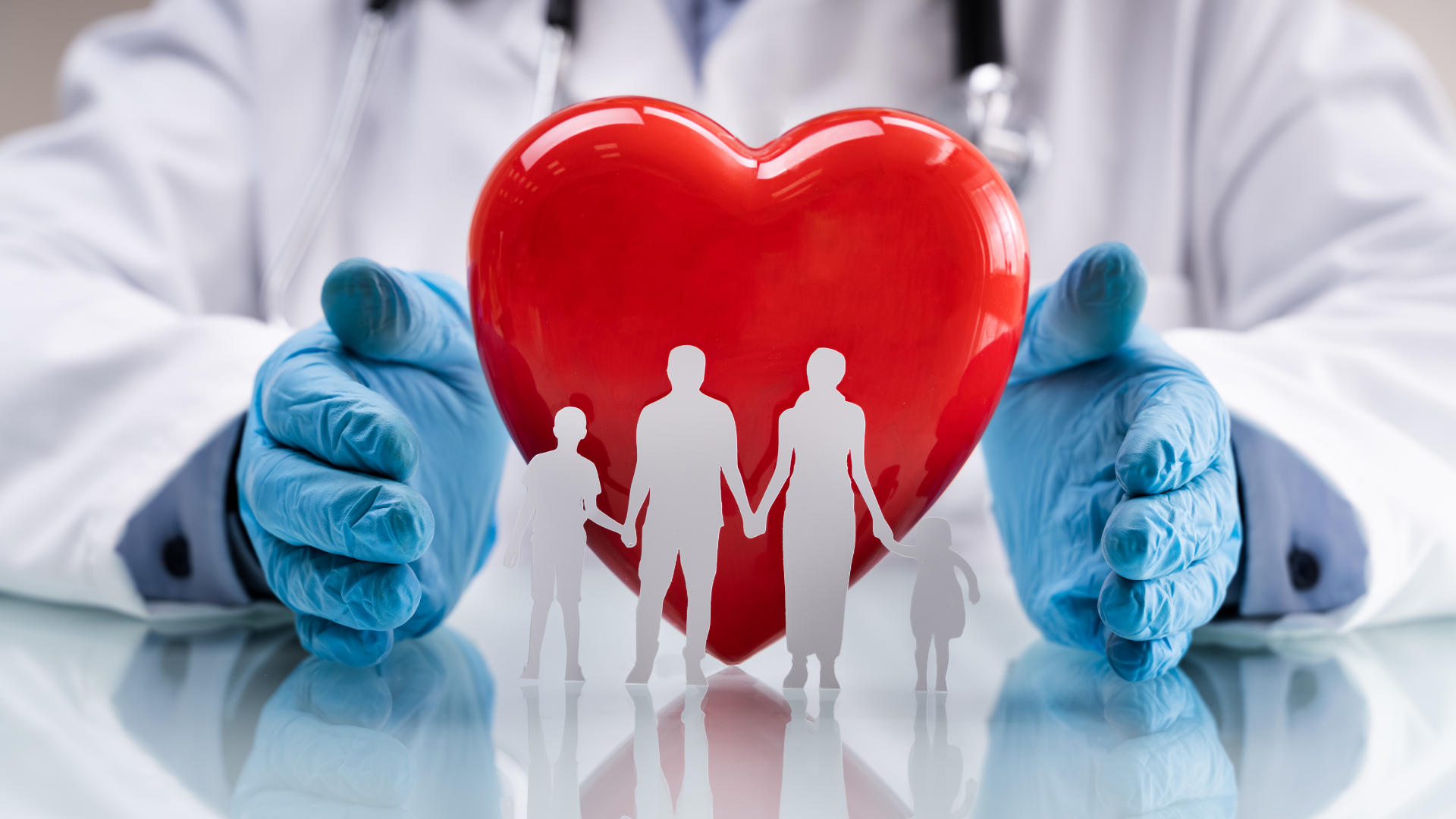