علم الكيمياء
تاريخ الكيمياء والعلماء المشاهير
التحاضير والتجارب الكيميائية
المخاطر والوقاية في الكيمياء
اخرى
مقالات متنوعة في علم الكيمياء
كيمياء عامة
الكيمياء التحليلية
مواضيع عامة في الكيمياء التحليلية
التحليل النوعي والكمي
التحليل الآلي (الطيفي)
طرق الفصل والتنقية
الكيمياء الحياتية
مواضيع عامة في الكيمياء الحياتية
الكاربوهيدرات
الاحماض الامينية والبروتينات
الانزيمات
الدهون
الاحماض النووية
الفيتامينات والمرافقات الانزيمية
الهرمونات
الكيمياء العضوية
مواضيع عامة في الكيمياء العضوية
الهايدروكاربونات
المركبات الوسطية وميكانيكيات التفاعلات العضوية
التشخيص العضوي
تجارب وتفاعلات في الكيمياء العضوية
الكيمياء الفيزيائية
مواضيع عامة في الكيمياء الفيزيائية
الكيمياء الحرارية
حركية التفاعلات الكيميائية
الكيمياء الكهربائية
الكيمياء اللاعضوية
مواضيع عامة في الكيمياء اللاعضوية
الجدول الدوري وخواص العناصر
نظريات التآصر الكيميائي
كيمياء العناصر الانتقالية ومركباتها المعقدة
مواضيع اخرى في الكيمياء
كيمياء النانو
الكيمياء السريرية
الكيمياء الطبية والدوائية
كيمياء الاغذية والنواتج الطبيعية
الكيمياء الجنائية
الكيمياء الصناعية
البترو كيمياويات
الكيمياء الخضراء
كيمياء البيئة
كيمياء البوليمرات
مواضيع عامة في الكيمياء الصناعية
الكيمياء الاشعاعية والنووية
Molecular Fluorescence
المؤلف:
LibreTexts Project
المصدر:
................
الجزء والصفحة:
.................
18-4-2020
2049
Molecular Fluorescence
When a molecule absorbs a photon in the ultraviolet or visible (UV VIS) region (180 - 780 nm), an electronic transition occurs within the molecule. This transition involves moving an electron from the singlet ground state to a singlet excited state. After excitation, the molecule will undergo de-excitation to regain its ground state electronic configuration. De-excitation of the molecule can occur in three distinct ways: by collisional deactivation (external conversion), fluorescence, or phosphorescence (Figure 1.1).
The first relaxation mechanism, collisional deactivation, occurs when the excited molecule transfers its excess energy to molecules with which it collides without photon emission. The second mechanism, fluorescence, involves the electron returning from the excited singlet state to the ground singlet state accompanied by the emission of a photon of lower energy (longer wavelength) than the absorbed photon; the energy loss is due to vibrational relaxation while in the excited state. When fluorescence is favored, it occurs within about 10-8 seconds after absorption. For reference, a picosecond is 10-9 seconds. Phosphorescence, the third route for de-excitation, occurs when the excited electron enters the lowest triplet state from the excited singlet state by intersystem crossing and subsequently emits a photon in returning from the lowest triplet state to the singlet ground state. These excited singlet-triplet and triplet- ground singlet transitions involve electron reversal (Figure 1.2), which is a low probability occurrence. Thus, the time between absorption and phosphorescence can be from 10-2 seconds to several minutes.
Figure 1.1: Jablonski diagram displaying excitation and de-excitation pathways of a molecule. λ0 indicates the incident wavelength that is absorbed by the molecule. Once the molecule reached the excited singlet state (S1) from the ground state singlet (S0), is can experience vibrational relaxation as seen in A. After relaxing to the lowest energy excited state, a molecule can de-excited by fluorescence as seen in path B. The fluorescence path emits a wavelength (λf) which is longer than the absorbed wavelength (λ0). The alternative, unfavored path moves along path C which involves intersystem crossing. Intersystem crossing involves a conversion from an excited singlet state(S1) to an excited triplet state (T1). After reaching the triplet state and experiencing vibrational relaxing, the molecule will phosphoresce by emitting an even shorter wavelength (λp) to reach the ground state singlet (S0).
Figure 1.2: Transition from Ground Singlet (A) to Excited Singlet (B) to Excited Triplet (C).
Since our interest involves fluorescence, a typical experimental arrangement is shown in Figure 1.3. This is used in either of two distinct ways. The first, which holds the excitation monochromator M1 fixed, and varies the emission monochromator M2, yields an emission spectrum (the wavelength distribution of light emitted by the excited singlet state). When generating an emission section, the intensity of the transmitted light (I) is measured and compared to the transmitted light's measured wavelength (λf). Alternately, M2 can be fixed and M1 varied; this procedure produces an excitation spectrum (a plot of fluorescence intensity as a function of excitation wavelength). The excitation spectrum can be produced by measuring the intensity of the transmitted light (I) and comparing it to the measured wavelength of the incident light (λ0). Often, the excitation spectrum of a pure compound has exactly the same profile as the absorption spectrum (Figure 1.1). The principles described by Figure 1.1 can be observed in the excitation and emission spectra. An example of these spectra can be seen in Figure 1.4.
Figure 1.3: Schematic Diagram of Fluorometer. M1 = excitation monochromator, M2 emission monochromator, Photo Multiplier Tube detector.
Figure 1.4: Excitation/Absorbance Spectrum (Red) coupled with an emission spectrum (Green). Each spectrum peaks at its
respective wavelength. Excitation and emission spectra should overlap and be mirror images of each other.
Coupling the above techniques with a relationship between fluorescence intensity and concentration would be exceptionally useful. Such a relationship can be derived from Beer's Law, which states that the fraction of light intensity transmitted by a sample is
where,
- I is the transmitted light intensity,
- I0 is the incident intensity,
- ε is the molar absorptivity at a given wavelength in units of L*mol-1*cm-1,
- b is the cell path in centimeters, and
- c is the concentration in moles per liter.
The fraction of absorbed light is:
from which it follows that the absolute amount of absorbed light is equal to:
The fluorescence intensity, F, is proportional to the amount of light absorbed and fluorescence quantum yield, Φ. Thus,
where k is a proportionality constant. If dilute solutions are used, so that less than 2% of the excitation energy is absorbed, the exponential term in Equation above can be approximated by the first two terms in the corresponding Taylor series expansion
hence fluorescence intensity is proportional to concentration, but only at low concentration:
الاكثر قراءة في التحليل الآلي (الطيفي)
اخر الاخبار
اخبار العتبة العباسية المقدسة
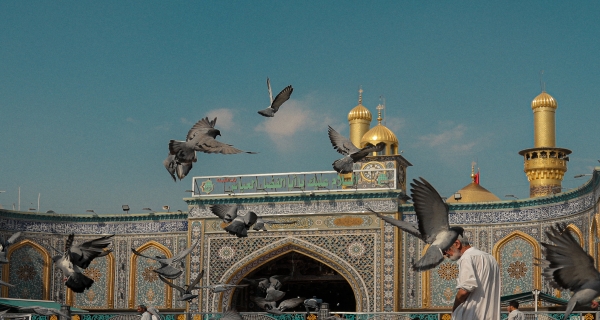
الآخبار الصحية
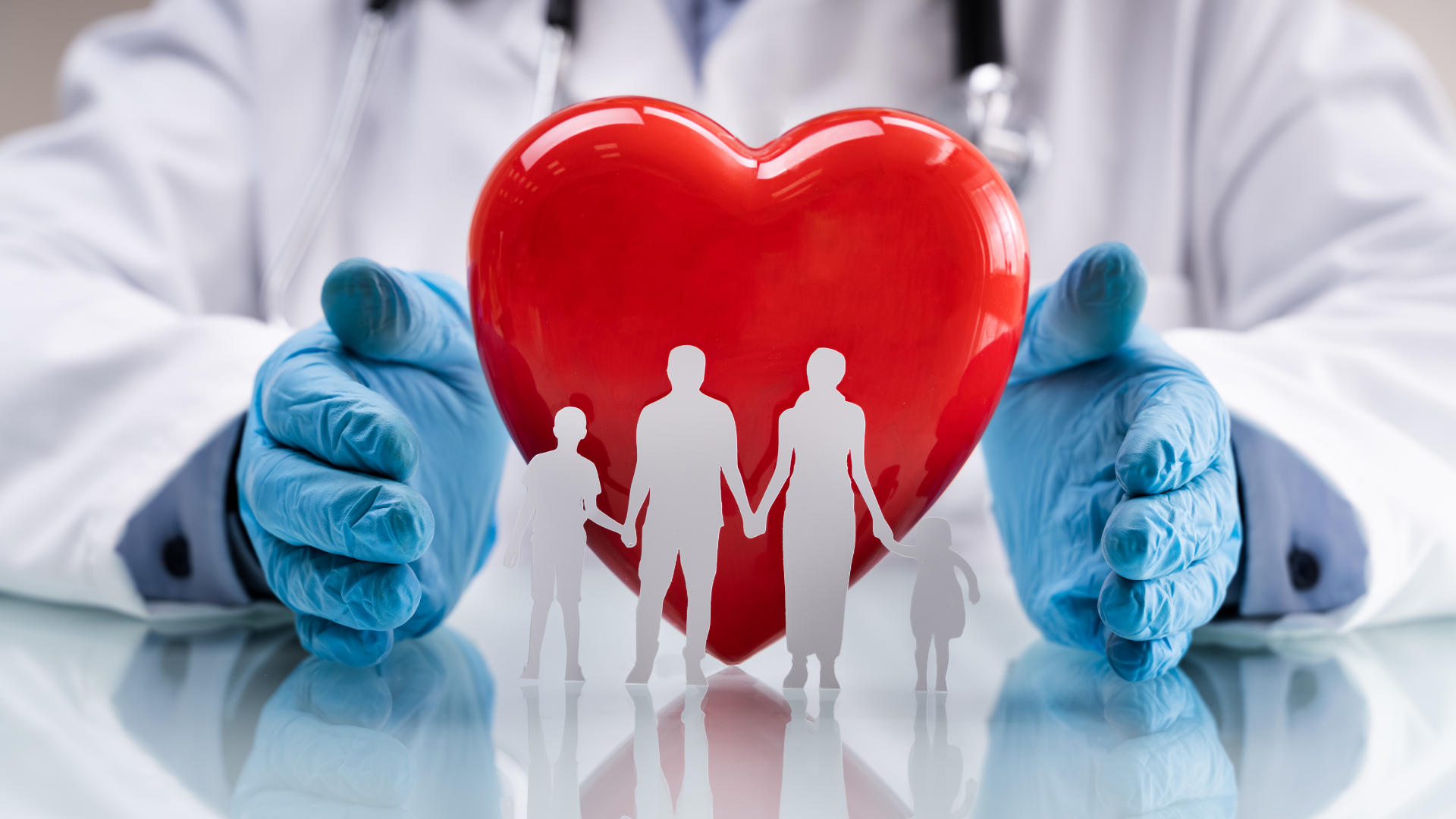